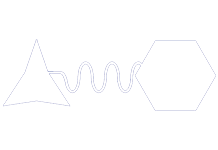
Targeted Protein Degraders
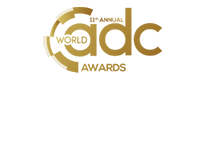
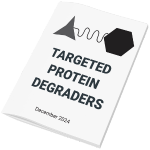
Download PDF
Mechanism of Targeted Protein Degradation | Targeted Protein Degraders: Paving the way for therapeutic revolution
Hijacking Protein Degradation Pathways | Evolution of Targeted Protein Degraders
Overview of current trials & developments in TPD space | How does one develop a TPD? | Business deals in TPD space
Developing and Optimizing Targeted Protein Degraders
David Fry* and Julien Dugal-Tessier
NJ Bio, 350 Carter Rd, Princeton, NJ 08540, U.S.A.
Last updated on 12th December 2024
What is Targeted Protein Degradation?
At the cellular level, many known mechanisms leading to diseases involve some type of protein interaction or imbalance. Most recently, dysregulation of protein degradation pathways has been associated to be a critical factor of many human diseases.1 This may include either lack of degradation or excessive degradation.2 Multi-omics analysis of various diseases like cancer has helped in identification of a variety of therapeutic protein targets. However, some of these target proteins, such as transcription factors and scaffold proteins, lack active binding pockets for small molecule inhibitors (SMI), limiting the design and development of drugs to target these proteins. It has also been difficult to target some therapeutically important proteins due to their broad active sites which are difficult to span with small molecules. 3
Targeted protein degradation (TPD) is a revolutionary therapeutic strategy that is refining the precepts of classical drug discovery. TPDs represent a new class of drugs4–8 which work by hijacking the endogenous protein quality control systems to produce therapeutic outcomes.9 These “two-headed” molecules usually involve a protein target binder at one end and an E3 ubiquitin ligase recruiter at the other, separated by a central linker.8 The result of this scaffold is forced proximity of the target protein to the E3 ligase, causing ubiquitin tagging for proteasomal destruction. Upon degradation, the TPD is released and able to target the POI again.10 (Figure 1). This strategy is based on target modulation guided by degradation of a protein of interest (POI) in the cell. This approach shows potential at developing therapeutic agents with improved resilience to point mutations and are potent even in the low nanomolar range.9 Rapid advancement in the field has led to expansion of such therapeutic degraders being studied extensively for applications ranging from oncology to antimicrobial resistance and beyond.
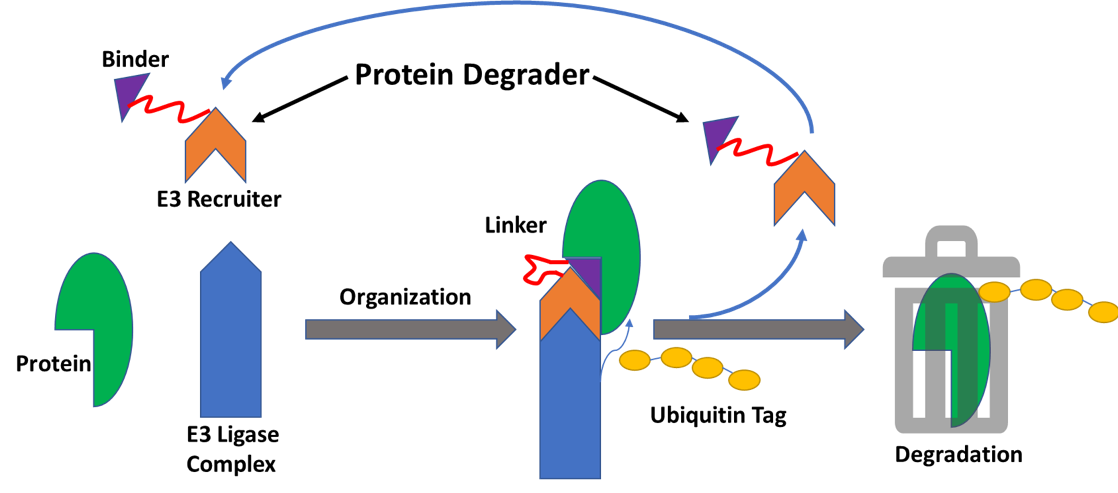
Targeted protein degraders: Paving the way for therapeutic revolution
Targeted protein degraders have extensive therapeutic scope due to their alluring potential to overcome resistance that has arisen to existing small-molecule inhibitors. By using small molecules for targeted protein degradation major proteins that were once considered ‘undruggable’, such as those with active sites that do not support binding of drug-like molecules, proteins with no active site, and some protein-protein interfaces are now potentially druggable. There are two major types of TPDs that leverage the ubiquitin-proteosome system (UPS), namely monofunctional degraders and heterobifunctional degraders. Monofunctional degraders directly interact with target proteins to induce degradation (e.g., some estrogen receptor degraders and androgen receptor degraders) or modulate target protein binding with E3 ligases (e.g., molecular glues). Heterobifunctional degraders, include chimeric compounds such as proteolysis-targeting chimeras (PROTACs).
TPDs offer benefits over traditional enzymatic inhibitors, as the binding site of the POI does not need to be continuously inhibited. The TPD platform can also allow expansion and reuse of currently available molecules. These can include molecules that lack specificity or have resistance.12 Further, because its action is catalytic13, these degraders require a smaller dosage and have a stronger sustained efficiency. All being said, TPDs come with their own range of challenges and limitations. These include a relatively large molecular weight, E3 ligase-related drug resistance and inability to degrade certain protein aggregates, which limits their development to a certain extent 14. Approaching early drug discovery and subsequent screening in the right way can limit these drawbacks and help develop a more practical and viable TPD for clinical use.
Hijacking Protein Degradation Pathways
Most generally, in eukaryotic systems, protein quality control is maintained by the UPS. This system is tightly regulated through a complex signalling pathway. It requires misfolded proteins to be tagged with ubiquitin to induce degradation. The UPS is one of the major pathways responsible for protein degradation in vivo and participates in nearly 80% of protein degradation occurring in the cell. The UPS offers scope of exploring a multitude of previously unexplored targets that can be employed for therapeutic intervention. 11 Apart from the UPS, lysosomal degradation pathway is another significant protein degradation pathway that is independent of the proteosome. This includes the endosome-lysosome pathway and the autophagy- lysosomal pathway. 14
Current advances in TPD design have brought about variations in the strategies of employing protein degradation mechanisms. This has led to different TPD drug classes, such as PROTACs15, SNIPERS (Specific and Non-genetic IAP-dependent Protein Erasers)16, Molecular glue (no linker) 17, AUTACs (Autophagy Targeting Chimeras)18, LYTACs (Lysosome targeting Chimeras)19, etc. The chimera approach has also moved beyond protein degradation to concepts such as RIBOTAC (RNA targets degraded by ribonuclease)20 and PhoRC (directed dephosphorylation of proteins)21. Additional approaches which are still in early development include use of photoactivation (PHOTACs), in-assembly of protein degraders (CLIPTACs) 22 and tag-based protein degraders (HyTag, SMASHTag and dTag).
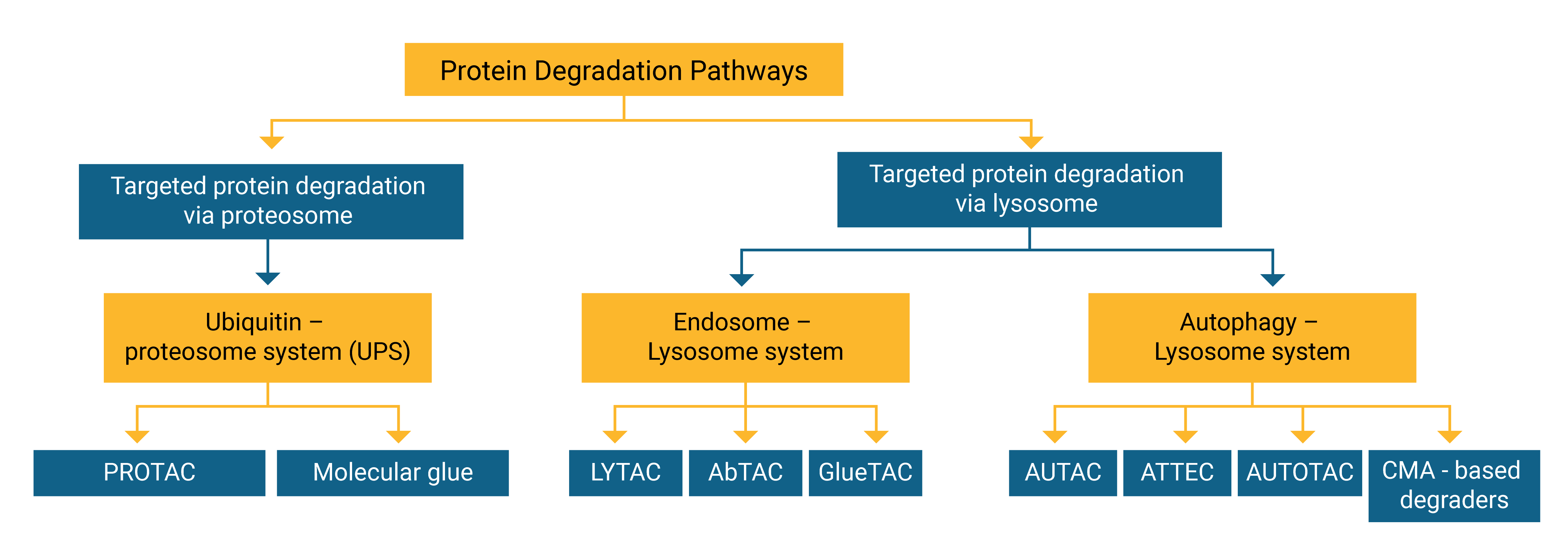
Evolution of Targeted Protein Degraders
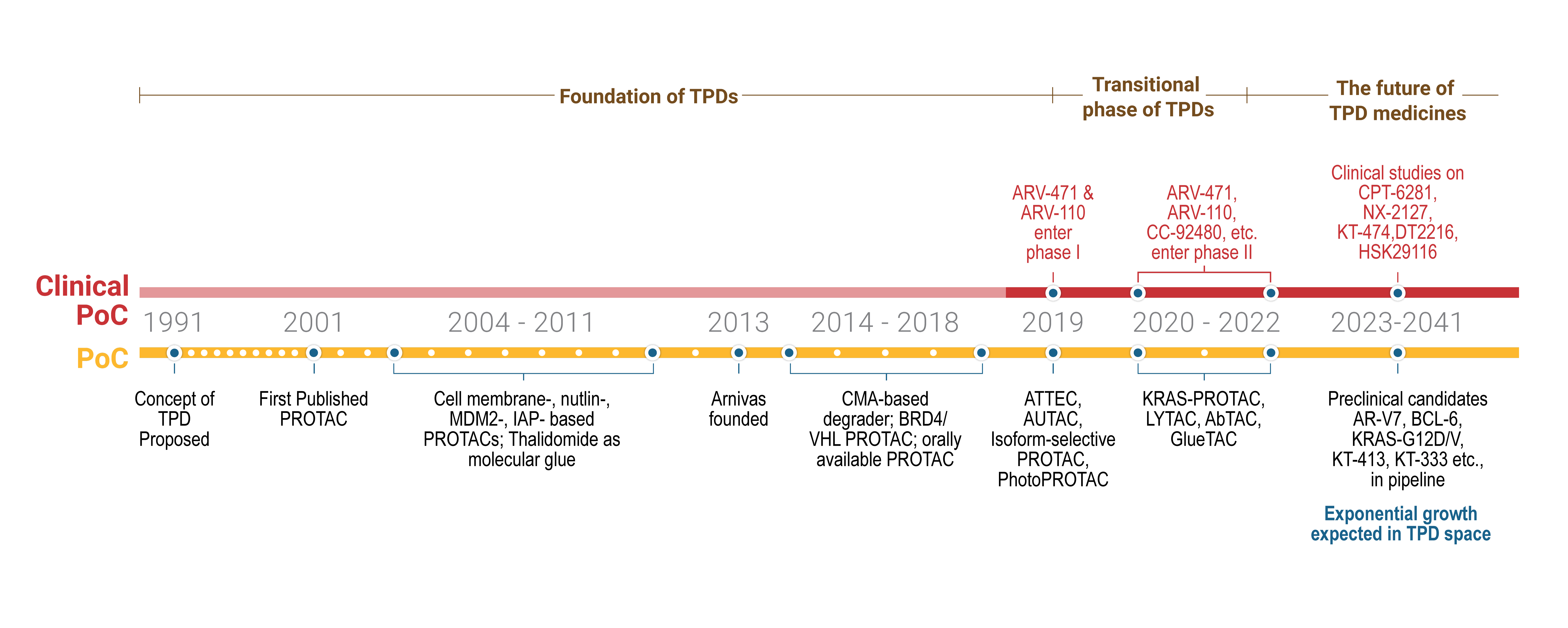
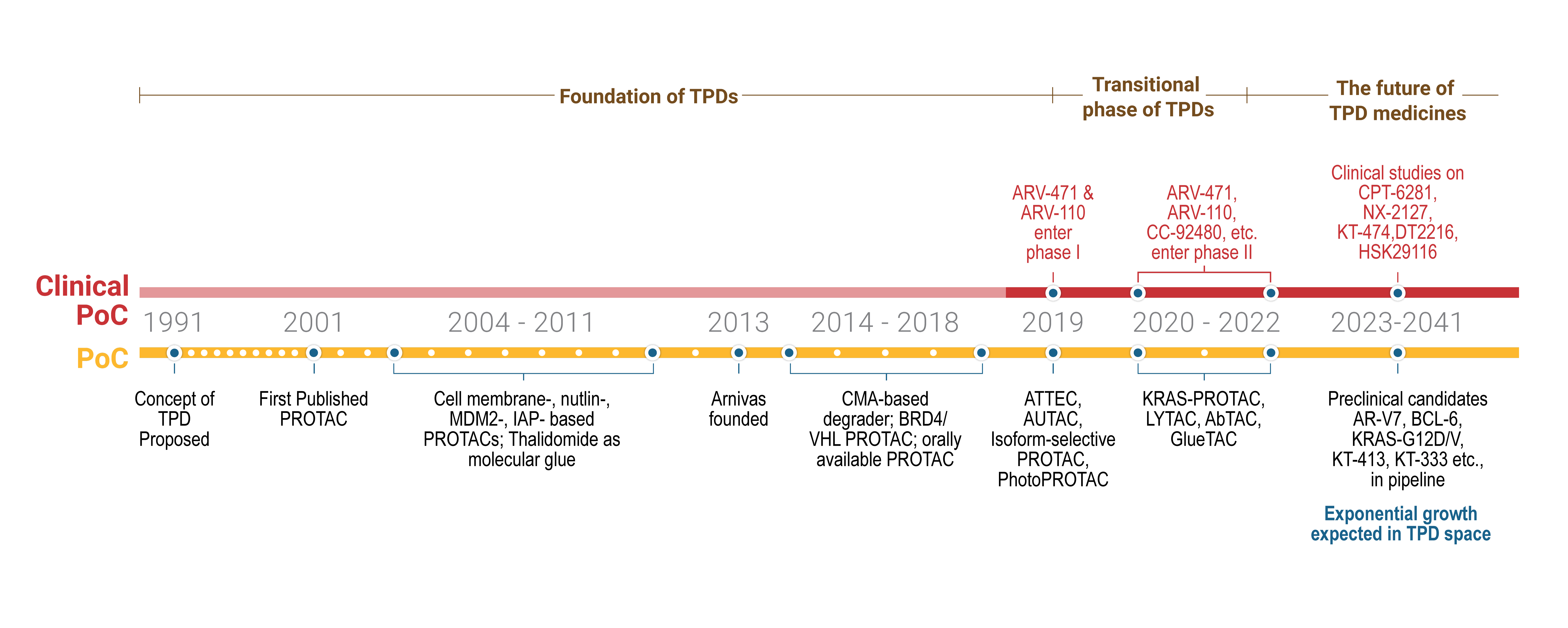
Figure 3: Timeline of TPD discoveries10
The first successful example of TPD was reported in 2001 from the labs of Sakamoto, Crews and Deshaies.24 These molecules were termed “PROTACs” and have been trademarked as such, thus the more general term targeted protein degradation (TPD) was developed. Since this landmark work, the field has steadily expanded, and there have now been many TPDs reported. In 2013, Arnivas Biopharmaceuticals began clinical development of their PROTAC candidates ARV-110 and ARV-471. Several other candidates from various pharmaceutical companies are currently in Phase I and Phase II trials or filed for IND approval. The current era of TPD can be considered as a translational phase, in which multiple protein degraders are entering clinical trials.10 The growing interest in the TPD space showcases the revolutionary effect this therapeutic modality is going to have in the future of medical science.
Overview of current clinical trials and developments in TPD space
Currently there are more than 130 TPD targets identified out of which around 20 have now entered clinical trials.10,25 The speed in discovery of new degradable targets have significantly surpassed the records of the previous 18 years and the degrader toolbox is growing rapidly.26
Many pharmaceutical companies have disclosed programmes that are in preclinical and clinical development. Start-up companies such as Arvinas, Nurix, C4 Therapeutics, Kymera Therapeutics, Cullgen, and Captor Therapeutics were the pioneers who focused on bringing the TPD strategy to market. In 2019, the first PROTAC candidates from Arnivas entered clinical testing; ARV-110 for metastatic castration-resistant prostate cancer (mCRPC) 27 and ARV-471 for ER-positive/HER2-negative breast cancer.25,28 These two TPD compounds have made it as far as Phase III and Phase II clinical trials respectively, providing proof of the therapeutic modality against cancer targets.3 They have demonstrated desirable safety, efficient dosage and clinical efficiency which highlight their potential viability in cancer treatment. Bristol Myers Squibb, Dialectic, C4 Therapeutics, Kymera, and Nurix also have clinical candidates being tested for the treatment of resistant cancers such as synovial sarcoma, B cell malignancies, multiple myeloma, and lymphoma.9 Recent advances in developing molecular glue compounds like CC-90009 and CC-92480 by Bristol Myer Squibb emphasize on the growing research around protein degradation as a therapeutic modality.10 Table 1 highlights the various potential targets in addition to AR and ER that have emerged in recent years viz. BCL-XL, IRAK4, STAT3, BTK, BRD9, etc.
Drug | Degrader Modality | Target | E3 Ligase | Company or Sponsor | Lead Indication | Phase of Development |
Pomalidomide (Pomalyst) | Molecular glue | IKZF1/ 3 | CRBN | Bristol Myers Squibb/ Celgene | Multiple myeloma | Approved (2013) |
Lenalidomide (Revlimid) | Molecular glue | IKZF1/ 3 | CRBN | Bristol Myers Squibb/ Celgene | Multiple myeloma | Approved (2005) |
Thalidomide (Thalomid) | Molecular glue | IKZF1/ 3 | CRBN | Bristol Myers Squibb/ Celgene | Multiple myeloma | Approved (1998) |
ARV-471 (Vepdegestrant ) | Heterobifunctional | ER | CRBN | Arvinas/ Pfizer | Breast cancer | Phase 3 (Fast-track designation by FDA) |
CC-220 | Molecular glue | IKZF1/ 3 | CRBN | Bristol Myers Squibb/ Celgene | Solid tumors | Phase 3 |
CC-92480 (Mezigdomide) | CELMoD | IKZF1/ 3 | CRBN | Bristol Myers Squibb/ Celgene | Multiple myeloma | Phase 3 |
ARV-110 (Bavdegalutamide) | Heterobifunctional | AR | CRBN | Arvinas/ Pfizer | mCRPC | Phase 2 |
ARV-766 | Heterobifunctional | AR | CRBN | Arvinas/ Novartis | mCRPC | Phase 2 |
KT-474 | Heterobifunctional | IRAK4 | Undisclosed | Kymera Therapeutics/ Sanofi | Immune-inflammatory diseases like hidradenitis suppurativa (HS), atopic dermatitis (AD) | Phase 2 |
CC-99282 | CELMoD | IKZF1/ 3 | CRBN | Bristol Myers Squibb/ Celgene | Lymphoma | Phase 2 |
CC-90009 | CELMoD | GSPT1 | CRBN | Bristol Myers Squibb/ Celgene | Acute myeloid leukemia | Phase 2 |
E7820 | CELMoD | RBM39 | Undisclosed | Eisai, Inc. | Solid tumors | Phase 2 |
GT20029 | PROTAC | AR | Undisclosed | Kintor Pharmaceuticals | Acne vulgaris, AGA | Phase 2 |
PRT3789 | Molecular glue | SMARCA2 | VHL | Prelude Therapeutics | SMARCA4 - del NSCLC | Phase 2 |
NX-2127 | Heterobifunctional | BTK, IKZF | CRBN | Nurix Therapeutics | B cell malignancies | Phase 1b |
HP518 | PROTAC | AR | Undisclosed | Hinova Pharmaceuticals | mCRPC | Phase 1a |
BGB-16673 | cDAC | BTK | Undisclosed | BeiGene | (R/R) CLL/SLL | Phase 1/2 (Fast-track designation) |
RMC-6291 | Molecular glue | KRASG12C | Undisclosed | Revolution Medicines | Solid tumors | Phase 1/2 |
ICP-490 | Molecular glue | IKZF1/ 3 | CRBN | InnoCare Pharma | Multiple myeloma | Phase 1/2 |
CFT-1946 | BiDAC | BRAF V600 | CRBN | C4 Therapeutics | NSCLC, Colorectral cancer, melanoma | Phase 1/2 |
CFT-7455 | CELMoD | IKZF1/ 3 | CRBN | C4 Therapeutics | Multiple myeloma and Non-Hodgkin's lymphoma | Phase 1/2 |
MRT-2359 | Molecular glue | GSPT1 | CRBN | Monte Rosa | Lung Cancer, Solid Tumor | Phase 1/2 |
CFT-8634 | BiDAC | BRD9 | CRBN | C4 Therapeutics | Synovial sarcoma and SMARCB1-null solid tumors | Phase 1/2 |
CG00149 | Heterobifunctional | TRK | Undisclosed | Cullgen | Solid tumors | Phase 1/2 |
RNK05047 | CMA-based degrader | BRD4 | CRBN | Ranok Therapeutics | DLBCL | Phase 1/2 |
DKY-709 | Molecular glue | IKZF2 | CRBN | Novartis | Solid tumors | Phase 1/1b |
NVP-DKY709 | Molecular glue | IKZF2 | CRBN | Novartis | Solid tumors | Phase 1/1b |
ARV-102 | Heterobifunctional | LRRK2 | Undisclosed | Arvinas | Neurological Diseases like Parkinson's and Progressive Supranuclear Palsy | Phase 1/1b |
NX-5948 | Chimeric degrader | BTK | CRBN | Nurix Therapeutics | B cell malignancies | Phase 1 (Fast-track designation by FDA) |
DT2216 | APTaD | BCL-XL | VHL | Dialectic Therapeutics/ University of Florida | (R/R) PTCL and CTCL | Phase 1 (Fast-track designation by FDA) |
NST-628 | Nondegrading Molecular glue | RAF-MEK | Undisclosed | Nested Therapeutics | Solid tumors | Phase 1 |
ORM-6151 | Dual-Precision Targeted Protein Degradation (TPD²) | CD33 | Undisclosed | Orum Therapeutics | Acute Myeloid Leukemia | Phase 1 |
FHD-609 | Heterobifunctional | BRD9 | Undisclosed | Foghorn Therapeutics | Synovial Carcinoma | Phase 1 |
FD-001 | Molecular glue | GSPT1 | Undisclosed | Chengdu Fendi Pharmaceutical Co., Ltd | Acute Myeloid Leukemia, Non-Hodgkin Lymphoma, Multiple Myeloma | Phase 1 |
GLB-002 | Molecular glue | IKZF1/3 | Undisclosed | GluBio Therapeutics | Relapsed or Refractory non-Hodgkin lymphoma (R/R NHL) | Phase 1 |
ORM-5029 | Dual Precision TPD² | GSPT1 | Undisclosed | Orum Therapeutics | HER2 Positive Solid Tumors | Phase 1 |
ST-00937 | Molecular glue | RBM39 | Undisclosed | Seed Therapeutics | Neoplasms | Phase 1 |
HSK29116 | PROTAC | BTK | Undisclosed | Haisco Pharmaceutical Group Co., Ltd. | B-Cell Lymphoma B-Cell Malignant Neoplasm | Phase 1 |
MRT-6160 | Molecular glue | VAV1 | Undisclosed | Monte Rosa Therapeutics | Multiple T-cell mediated autoimmune and inflammatory diseases | Phase 1 |
AR-LDD (CC-94676) | Heterobifunctional | AR | CRBN | Bristol Myers Squibb | mCRPC | Phase 1 |
KT-413 | Heterobifunctional | IRAK4 | Undisclosed | Kymera Therapeutics | MYD88-mutant B-cell lymphomas | Phase 1 |
KT-333 | Heterobifunctional | STAT3 | Undisclosed | Kymera Therapeutics | CTCL, NHL, PTCL | Phase 1 |
AC0699 | Heterobifunctional | ER | Undisclosed | Accutar Biotech | Breast cancer | Phase 1 |
AC0682 | Chimeric degrader | Erα | CRBN | Accutar Biotech | ER+ Breast Cancer | Phase 1 |
AC0176 | Chimeric degrader | AR | Undisclosed | Accutar Biotech | mCRPC | Phase 1 |
AC0676 | Chimeric degrader | BTK | Undisclosed | Accutar Biotech | Hematology & Oncology Indications | Phase 1 |
ASP3082 | Heterobifunctional | KRAS G12D | Undisclosed | Astellas | Pancreatic, Colorectal and Lung cancer with KRAS G12D mutation | Phase 1 |
ASP4396 | Heterobifunctional | KRAS G12D | Undisclosed | Astellas | Colorectal and Pancreatic Cancer | Phase 1 |
KT-253 | Heterobifunctional | MDM2 | Undisclosed | Kymera Therapeutics | ALL, AML, Solid Tumors | Phase 1 |
GT-919 | Molecular glue | IKZF1/ 3 | Undisclosed | Gluetacs Therapeutics | Multiple myeloma | Phase 1 |
BTX-1188 | Molecular glue | IKZF1/ 3 | CRBN | Biotheryx | Hematologic and Solid malignacies | Phase 1 |
BAY-2666605 | Molecular glue | PDE3A/ SLFN12 | Undisclosed | Bayer | Melanoma | Phase 1 |
CC-122 | Molecular glue | IKZF1/ 3; ZFP91 | CRBN | Bristol Myers Squibb/ Celgene | Various Cancer | Phase 1 |
PLX-4545 | Molecular glue | IKZF2 | Undisclosed | Plexium, Inc. | Various Cancer | Phase 1 |
ARV-393 | PROTAC | BCL6 | Undisclosed | Arvinas | Non Hodgkin Lymphoma and Angioimmunoblastic T-cell Lymphoma | Phase 1 |
SP-3164 | Molecular glue | IKZF1/3 | CRBN | Salarius Pharmaceuticals | NHL | Early Phase 1 |
PROTAC: Proteolysis-targeting chimera; APTaD: Antiapoptotic Protein Targeted Degradation; BiDAC:Bifunctional degradation activating compounds; cDAC: Chimeric Degradation Activation Compounds; CELMoD:Cereblon E3 Ligase Modulatory Drugs; mCRPC: Metastatic Castration-Resistant Prostate Cancer; (R/R) PTCL and CTCL: Relapsed or Refractory Peripheral T-cell Lymphoma and Cutaneous T-cell Lymphoma; NSCLC: Non-small cell lung cancer; NHL: Non-Hodgkin’s Lymphoma; AGA: Androgenetic Alopecia; ALL: Acute Lymphocytic Leukemia, AML: Acute Myelocytic Leukemia, DLBCL: Diffuse Large B-cell Lymphoma, CMA-based degrader: Chaperone mediated autophapy – based degradation, CLL: Chronic Lymphocytic Leukemia, SLL: Small Lymphocytic Lymphoma
How does one develop a TPD?
While developing a TPD the fundamental approach would be to design a synthetic low-molecular weight molecule that can alter the specificity of cellular machinery which is involved in protein degradation.11 Fig. 4 highlights the basic roadmap of designing therapeutic protein degraders.
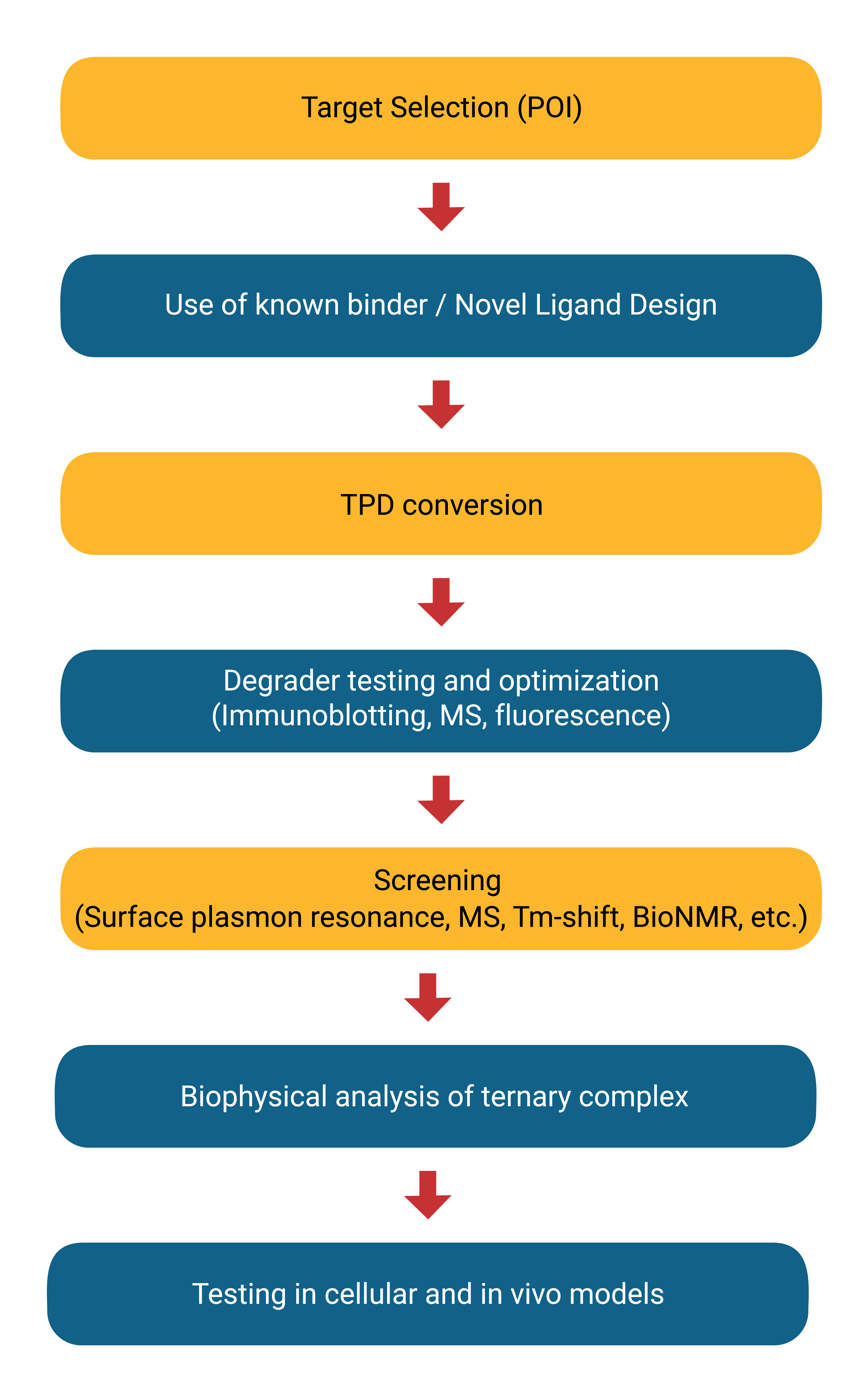
Figure 4: Potential roadmap for developing protein degraders
It would be fitting to address the question of developing a TPD by considering the three components of a TPD and their roles: binder to the target protein, the E3 recruiter, and the linker joining these pharmacophores.
The Target Protein Binder
The target protein is one whose removal will alleviate the disease condition. Many of these proteins have been considered for conventional drug discovery efforts. Thus, the identification of a ligand for the target protein will fall into two classes: known and unknown binders.
Known binders are molecules that are approved or ones that have clinical or preclinical data. If the protein of interest has an approved drug, making a TPD could improve that drug by increasing selectivity, bioavailability and/or eliminating resistance. If the protein of interest does not have an approved drug but has known binders, these could be rejuvenated through a TPD program.
The second class includes proteins for which a ligand/binder has yet to be discovered. This would necessitate a hit discovery program followed by a lead generation TPD program.
Since kinases are known to have effective inhibitors which can be modified to connect linkers, they are preferred POIs for TPDs. Reported studies show 54 kinases that can be degraded using TPD technology. Kinases also have deep binding pocket which can promote the binding of a TPD.26 Most protein kinases however lack a suitable active site. However, in targeted protein degradation, as the ligand of the POI does not need to mandatorily bind at the active site of the POI, this problem is surpassed.
Currently most lead discovery efforts involve screening a compound library and rationally designing a preliminary scaffold.29 Screening for a compound where the only requirement is binding would be best done by a biophysical method since an activity-based screen would miss the compounds that do not have inhibitory activity. Such biophysical screening methods include surface plasmon resonance (or a version thereof), mass spectrometry, Tm-shift, fluorescence polarization, calorimetry, X-ray crystallography and BioNMR spectroscopy. Most of these methods have been adapted for high-throughput robotic screening. The speed and reduced volumes of such screening usually result in a significant number of false positives. These false positives can lead to lengthy medicinal chemistry campaigns for hits that cannot be converted to leads. BioNMR spectroscopy and X-ray crystallography are lower throughput techniques but are gold standards for verifying binding. BioNMR is also the only method that can provide reliable proof of non-binding because it measures binding in solution.
The E3 Ubiquitin Recruiter
With over 600 E3 ligases in the human cell30, how does one select the proper E3 for a particular TPD? First, ubiquitination serves various purposes, not just tagging for proteolysis. Current studies identify 270 of the known ubiquitin ligases as being involved in the ubiquitin-proteosome system, and of those only 10 are very widely expressed, while the others have specific tissue-distribution profiles.19 Therefore, it is critical to verify E3 function, and match expression to the location being targeted but this could also be used to increase specificity of the E3 ligase only towards the tissue of interest.
Expression of E3 ligase varies with tissue type, tumor type, cellular compartment, or cell state. Thus, employing E3 ligases with a certain specific expression profile would help in the development of a specific TPD for precision medicine.2 In designing a viable TPD a crucial step is the identification of suitable ligase, for which the following points should be taken into consideration; (i) Not every ligase in suitable for TPD, (ii) Not every ligase/ target pair is compatible, (iii) Productive ternary complex formation drives TPD action. Hence, this step requires optimization at various levels.11
To date, the choice of E3 ligases has been dictated by whether an associated small molecule binder has been available and not because the optimal E3 ligase is being targeted. The vast majority of reported TPDs have utilized two E3 systems, identified by their target-protein recognition component – VHL (Von Hippel–Lindau tumor suppressor) and CBRN (Cereblon) – for both of which potent small molecule ligands are known. Other E3 ligases that have been used in TPDs include MDM2, cIAP, KEAP1, DCAF15, and DCAF16.
Involvement of new E3 ligases, exploration of other known ligands for established E3 recognition domains, and discovery of new ligands for yet untried E3 ligases are the obvious areas of high interest in the development of TPDs. The choice of E3 can endow a TPD with the critical attributes to make it a successful drug.30 However, with so many E3 ligases to choose from, it is helpful to have a rational framework to consider E3 options.31 E3 ligases can be divided into three classes, based on their target-recognition domain and ubiquitin transfer mechanism: RING domain (transfers ubiquitin directly), HECT domain (transfers ubiquitin via a thioester intermediate), and RBR (has a RING domain, but uses a thioester intermediate). The RING domain class can be further divided into those involving a single protein (such as MDM2 and the IAP family), and those involving a multi-subunit complex (such as VHL, CBRN, KEAP1, and the DCAFs). The multi-subunit systems are referred to as “Cullin-RING E3 ubiquitin ligases” (CRL) and have a generic arrangement that sequentially links a target protein recognition domain to one or more adaptor proteins, then to a Cullin domain, and then to a RING-Box (RBX) domain (Figure 5).
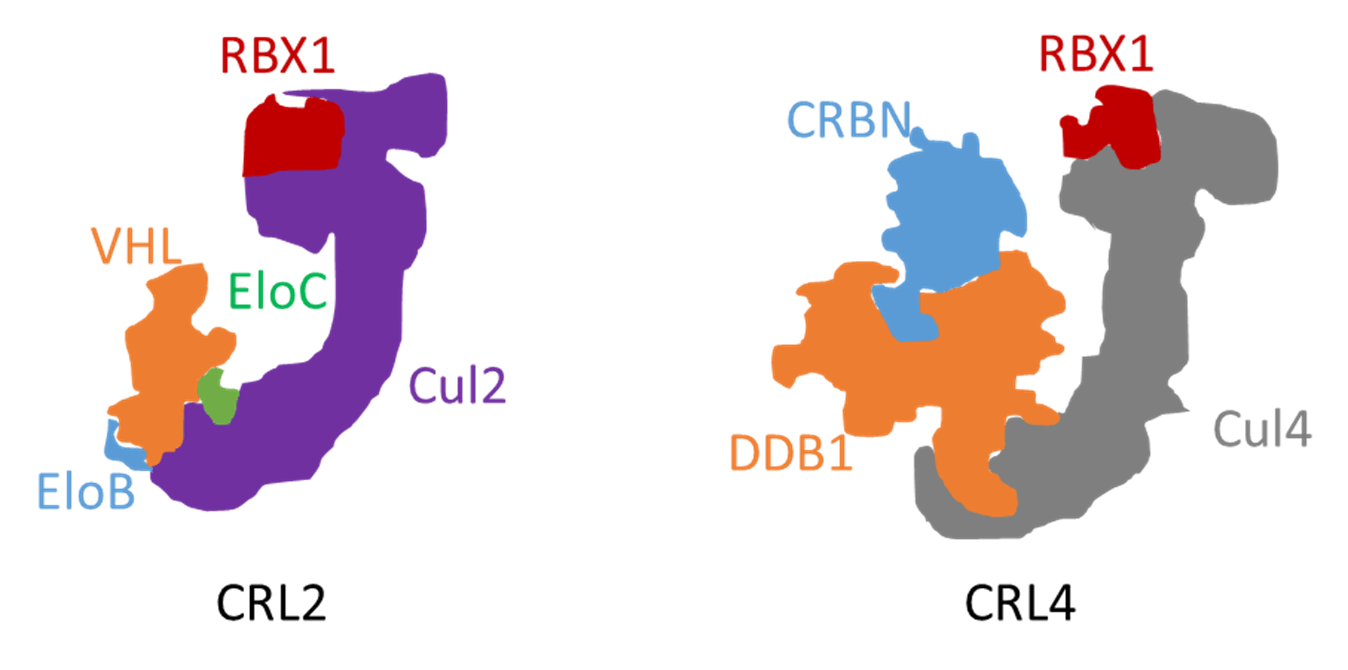
Figure 5: Depiction of Cullin-Ring E3 Ubiquitin Ligases (CRL) for the common VHL and CRBN type TPDs.
There are various peptide and small-molecule inhibitors known for VHL but reported TPDs utilizing this E3 ligase have almost exclusively employed one particularly potent small molecule which has come to be known as VH-298. Similarly, there are many TPDs reported utilizing CBRN, but almost all of them employ thalidomide or a close derivative as the E3 binder. TPDs based on MDM2 have so far largely utilized the small-molecule inhibitors called Nutlins, despite the existence of a wide variety of other potent small molecule inhibitors. TPDs based on cIAP have employed only a limited number of peptide-like inhibitors such as bestatin. There are however numerous drug-like inhibitors known for the IAP family, possessing many unique characteristics, such as selectivity for the BIR2 or BIR3 sub-domains which mediate ligand binding. As for more recently exploited E3 ligases, a TPD utilizing KEAP1 has been reported using a peptide as the binder, however there are now small drug-like inhibitors available for KEAP1.
To find a ligand for a new E3 ligase, the first step could be to examine the sequence of E3 ligases and see if it contains a domain that is known to support binding of small molecules.30 WDR domains are present in numerous E3 ligases, and these domains have been shown to bind small molecule ligands with nanomolar affinity. The same combination is true for Kelch domains and bromodomains. SPRY and WW domains are found in certain E3 ligases, and these domains have been shown to bind reasonably small linear and cyclic peptides. All these domains suggest that they could support binding of drug-like molecules and be applied to a TPD program.
Even though TPDs are still being developed, resistant mechanisms to these novel therapeutics have already surfaced. It is possible for mutation to take place in tumor cells to evade degraders that rely on non-essential ligases.10 The treatment fails to eliminate tumor cells, mostly due to downregulation of the E3 ligase or surviving cells carrying gene alterations on CRBN and VHL complexes. Also, some POIs may not be effectively degraded using CRBN or VHL. Another pressing issue in TPD development includes inducing ternary complex formation. Chimeric small molecules need to form ternary complex between E3 ligase and protein which persists long enough for E3 ligase to ubiquitinate its substrate. 2 Thus, it becomes important to expand the range of E3 ligases that can bypass these issues. Progenra, Inc., focused on novel membrane-targeted ubiquitin ligases by designing TPDs with promiscuous kinase inhibitors that degrade several kinases unlike the traditional TPDs.
The Linker
Currently, the linker is the most under-exploited TPD component since the choice of E3 ligands is limited and fixed. The linker must be properly selected to allow the TPD to function. From the few available X-ray structures of TPDs bound in a ternary complex 32–35 it appears that the target-binder and the E3 recruiter portions bind in the same manner as they do as standalone components. Even the available attachment points for the linker may be constrained by the way the target and E3 protein surround their respective binders. The linker usually is the only component of the platform that can be optimized for affinity, degradation efficiency, selectivity, and pharmacokinetic properties.
It is clear from X-ray structures that the TPD linker is not dumbbell-shaped, with the linker simply serving as a flexible connector between the target protein and the E3. Rather, the linker is folded into a particular conformation, and interacts with both the target protein and the E3. This pulls the two proteins into proximity leading to novel inter-protein contacts. The new contacts are essential but cannot be predicted without structural information. In fact, for two proteins which would normally never encounter each other, it is surprising how willing they are to interact. Preliminary data indicate that the inter-protein and linker-protein interactions appear to be opportunistic as they involve mostly hydrogen bonding and some moderate hydrophobic clustering, but not shape recognition or significant penetration. However, within the hydrogen bonds formed, there is a trend towards capping the termini of helices, and this may suggest that longer-range effects, such as pole orientations, are influencing how the complex forms. BioNMR is a rapid method for providing experimental information on the intermolecular contacts that are made and guiding rational design of TPDs.
How should one choose and optimize a TPD linker? It should first be stated that there are compact TPDs that have been called “molecular glues”, where a single organic scaffold brings together a target protein and a ubiquitin ligase without the need for what would be considered a “linker”. Ultimately, the target protein and E3 ligase must be brought together in a way that orients them properly for the ligase to catalytically attach a ubiquitin to a lysine on the protein of interest.
Critical attributes that can be measured include degradation efficiency and druglikeness. For example, a project could start with an established set of 25 linkers consisting of methylene chains, polyethylene glycol chains, and three others with more rigidity, each varied in carbon atom length. These could be attached to the target and E3 binders at various attachment points. These candidates could then be ranked by comparing their degradation efficiencies. At this point, structural guidance would become very valuable since a hit structure has been found and it would need to be rationally developed into a lead molecule. Modeling the ternary complex is an option but can be imprecise without structural information (i.e. X-ray crystallography). However, BioNMR is an alternative where one can gain speed by obtaining information that is at a lower resolution but can still improve the computational model. Through selective labeling of the target protein, the E3, and the TPD, BioNMR can indicate which regions of these components are participating in new interactions and can provide information on the bound conformation of the TPD. This data can be fed into modeling efforts to understand and visualize the ternary complex. BioNMR profiles can also be correlated with activity profiles to understand which attributes and overall structural consequences of the TPD are beneficial. With a better model, improved versions of the TPD can then be designed and increase confidence in the optimization campaign.
Another important attribute to consider is selectivity. It has been shown that the TPD context can endow target selectivity even if using a non-selective target protein binder.15 It is hard to predict what off-target effects to expect, but certain binder classes have clear selectivity issues. Structural information correlated to selectivity can help guide medicinal chemistry efforts toward compounds with enhanced specificity.36
The other set of attributes which must be optimized fall within the ADMET (absorption, distribution, metabolism, excretion, and toxicity) and pharmacokinetics properties. Druglikeness is a critical issue for TPDs because they tend to be large flexible molecules, which hampers their use as small molecule therapeutics.37 As stated, the front and back portions of the TPD are constrained by their necessary interactions with the target and E3 respectively, so any physiochemical liabilities they carry must be offset by the linker. To guide linker optimization, other key properties can be calculated, or experimentally measured, such as aqueous solubility, octanol/water partition coefficient (log P), and membrane permeability.38 Ultimately, the behaviors of TPDs that are serious drug candidates must further be assessed with regard to bioavailability, kinetics, metabolism and clearance. Despite their large size, it has been possible to develop TPDs that perform acceptably with regards to small molecule pharmacology. The clearest proof is the entry of two TPDs into phase II of clinical trials.
Larger issues such as toxicity are difficult to predict, but one obvious concern is that hijacking an E3 ligase to degrade a disease target will also degrade the protein in non-disease cells, and this may lead to unwanted side effects. One newly emerging idea, in which the linker plays a key role, is the attachment of antibodies to TPDs to target them to specific cells to avoid non-disease cell toxicity.39 Generally, the only portion of a TPD with accessible space for attaching such an appendage is the linker. In fact, a second linker leading to the antibody will almost surely be required. This reinforces the need for structural information, with regards to the optimal attachment point, length, rigidity, and chemotype of the second linker.
Business Deals in the Targeted Protein Degradation (TPD) Space
The significant rise in the number of TPDs entering clinical trials, along with favorable preclinical data demonstrating their superior efficacy and tolerability, has contributed to growing investments from both public and private sectors over the past five years. Lucrative partnerships and licensing agreements in oncology research, along with collaborations extending to non-cancer, immune-related, and neurological targets, highlight the growing interest in this modality across various disease indications. From a $135 million license agreement between Roche and Vividion Therapeutics in May 2020 to a $1.46 billion collaboration and license agreement between Novo Nordisk and Neomorph in February 2024, these TPD deals cover a wide spectrum, ranging from discovery to clinical trials. PROTAC degraders and molecular glues remain the most popular degrader modalities in TPD deals. Table 2 summarizes the competitive deal-making landscape, showcasing key partnerships and strategic collaborations in the TPD space since 2019. According to GlobalData’s statistics, venture financing in the TPD sector has seen a more than 2000% increase in recent years, growing from $33 million in 2017 to $707 million in 2022. Table 3 provides a summary of key venture capital funding events and successful IPOs in the TPD space since 2018.
Table 2: Key TPD Licensing Agreements and Collaborations between 2019 – 2023
Partners | Degrader Modality | Asset | Molecular Target | Deal Type | Date | Phase of Development | Deal value | Reference links |
Pulmatrix & Cullgen | Targeted Protein Degradation | PUR3100, and other development candidates based on iSPERSE™ technology | Undisclosed | Merger Agreement | Nov-24 | Phase 1 | $65 Million (Combined) | See Press Release |
Novartis & Monte Rosa Therapeutics | Molecualar Glue Degrader | MRT-6160 | VAV-1 | Global Exclusive Development & Commercialization License Agreement | Oct-24 | Phase 1 | $150 Million upfront; ~$2.1 Billion in future milestone payments; tiered royalties | See Press Release |
Pfizer & Triana | Molecualar Glue Degrader | Undisclosed | Undisclosed | Strategic Collaboration and Licensing Agreement | Oct-24 | Discovery | Upfront payment of $49 Million and to receive future milestone payments exceeding $1.5 Billion as well as tiered royalties | See Press Release |
Biogen & Neomorph | Molecular Glue Degrader | Neomorph's proprietary molecular glue discovery platform | Undisclosed | Research Collaboartion | Oct-24 | Discovery | ~$1.45 Billion | See Press Release |
SEED Therapeutics & Eisai Co., Ltd. | Molecular Glue Degrader | Undisclosed | Undisclosed | Strategic Research Collaboration | Aug-24 | Discovery | $1.5 Billion plus tiered royalties | See Press Release |
Astrazeneca & Pinetree Therapeutics | Targeted Protein Degrader | Undisclosed EGFR Degrader | Undisclosed | Exclusive option & global license agreement | Jul-24 | Preclinical | $45 Million upfront, over $500 Million in future milestone payments as well as tiered royalties | See Press Release |
Takeda & Degron Therapeutics | Glue Degraders | GlueXplorer® Platform | Undisclosed | Collaboration & Exclusive License Agreement | May-24 | Discovery | ~$1.2 Billion | See Press Release |
Novartis AG & Arnivas | PROTAC® | ARV-766 | AR | License agreement | Apr-24 | PII | $150 Million upfront; $180 Million potential milestone payments | See Press Release |
Merck KGaA & C4 Therapeutics | Targeted Protein Degraders | Undisclosed | Undisclosed | License & Collaboration Agreement | Mar-24 | Discovery | $16 Million upfront; up to $ 740 Million in potential milestone payments | See Press Release |
Bristol Myers Squibb & VantAI | Molecular Glues | Undisclosed | Undisclosed | Strategic Collaboration | Feb-24 | Discovery | $674 Million plus tiered royalties | See Press Release |
Novo Nordisk & Neomorph | Molcular Glue Degraders | Propietary Glue Discovery Platform | Undisclosed | Collaboration & License Agreement | Feb-24 | Discovery | $1.46 Billion | See Press Release |
Roche and Monte Rosa | Molecular Glue Degraders | QuEEN™ discovery engine | Undisclosed | Strategic collaboration & Licensing agreement | Oct-23 | Discovery | $50 Million upfront; potential future payments exceeding $2 Billion; tiered royalties | See Press Release |
Genentech & Orionis Biosciences | Molecular Glue Degraders | Allo-Glue™ | Undisclosed | Multi-year Collaboration | Sep-23 | Discovery | $47 Million upfront; ~$2 Billion in milestone payments | See Press Release |
Astellas and PeptiDream | Undisclosed | PDPS (Peptide Discovery Platform System) technology | Undisclosed | Research collaboration & License agreement | Jul-23 | Discovery | ¥3.0 Billion upfront; up to ¥20.6 Billion in milestone payments per target; single-digit percent royalty payments | See Press Release |
Astellas and Cullgen | Undisclosed | uSMITE™ targeted protein degradation platform | Undisclosed | Research collaboration & License agreement | Jun-23 | Discovery | $35 Million upfront; $85 Million license option | See Press Release |
Merck & Proxygen | Molecular Glue Degraders | Undisclosed | Undisclosed | Multi-year research collaboration & License Agreement | Apr-23 | Discovery | $2.55 Billion | See Press Release |
Incyte and Biotheryx | Molecular glue degraders | PRODEGY platform | Undisclosed | Research collaboration & License agreement | Apr-23 | Discovery | $7 Million upfront, $ 6 Million in R&D funding, $347 Million in milestone payments | See Press Release |
Blueprint Medicines & Proteovant Therapeutics | Undisclosed | TPD Platform | Undisclosed | Strategic collaboration | Apr-23 | Discovery | $20 Million upfront plus up to $632 Million in milestone payments; tiered royalties | See Press Release |
Bristol Myers Squibb and Evotec | Molecular glue based pipeline | Undisclosed | Undisclosed | Stategic Partnership | Mar-23 | Discovery | $75 Million | See Press Release |
Merck & Proxygen | Molecular Glue Degraders | Undisclosed | Undisclosed | Strategic Multi-year Research Collaboration and License Agreement | Jun-22 | Discovery | $554 Million plus royalties | See Press Release |
Merck and Amphista | Small molecule protein degraders | Undisclosed | Undisclosed | Strategic collaboration | May-22 | Discovery | €893.5 Million ; mid-single digit range royalties | See Press Release |
Bristol Myers Squibb and Amphista | Undisclosed | Eclipsys™ targeted protein degradation platform | Undisclosed | Strategic collaboration | May-22 | Discovery | $30 Million upfront; up to $1.25 Million in milestone payments; royalties | See Press Release |
Bristol Myers Squibb and Evotec | Molecular glue degraders | Undisclosed | Undisclosed | Strategic partnership (8-year extension and expansion of collaboration) | May-22 | Discovery | $200 Million upfront; milestone payments with deal potential up to $5 Billion | See Press Release |
Amgen and Plexium | Molecular glues/ Monovalent degraders | Undisclosed | Undisclosed | Collaboration and License agreement | Feb-22 | Discovery | $500 Million | See Press Release |
Novartis and Dunad | Monovalent degrader | Undisclosed | Undisclosed | Collaboration and License agreement | Nov-21 | Discovery | $24 Million* upfront; up to $1.3 Billion in future milestone payments and royalties | See Press Release |
Pfizer and Arnivas | PROTAC | ARV-471 | ER | Collaboration | Jul-21 | Phase II | $650 M upfront; $350 M equity investment; up to $400 M in milestone payments | See Press Release |
Sanofi and Kymera Therapeutics | Bivalent degrader | KT-474 | IRAK4 | Strategic collaboration | Jul-20 | Phase I | $150 Million upfront; up to $ 2 Billion in potential milestone payments; royalties | See Press Release |
Roche and Vividion Therapeutics | E3 ligases | Undisclosed | Undisclosed | License agreement | May-20 | Discovery | $135 Million | See Press Release |
Vertex Pharmaceuticals and Kymera Therapeutics | Undisclosed | Undisclosed | Undisclosed | Strategic collaboration | May-19 | Discovery | $70 Million* upfront; up to $1 Billion in potential future milestone payments and royalties | See Press Release |
Bayer and Arnivas | PROTAC | Undisclosed | Undisclosed | Collaboration | May-19 | Discovery | $60 Million* upfront; up to $ 685 Million in milestone payments; commercial royalties | See Press Release |
* Includes Equity Investment
Table 3: Protein Degraders Venture Capital Funding and IPOs
Company | Degrader Modality | Asset(s) | Funding Event | Date | Phase of Lead Asset at Time of Deal | Deal value |
PhoreMost | Molecular Glue | GlueSEEKER™ | Series B | Sep-24 | Preclinical | $12 million |
SEED Therapeutics | TPD | RBM39, undisclosed Tau degrader, Proprietary TPD Platform | Series A-3 | Aug-24 | Preclinical | $24 million |
Pinetree Therapeutics | TPD | AbReptor™ | Series A | Jul-24 | Discovery | $17 million |
Rapafusyn Pharmaceuticals | Non-degrading Molecular Glue | RapaGlue™ | Series A | Jun-24 | Discovery | $28 Million |
Monte Rosa Therapeutics | Molecular glue | Undisclosed | Underwritten Public Offering | May-24 | Preclinical | $100 million |
Lycia Therapeutics | LYTAC | Undisclosed | Series C | May-24 | N/A | $106.6 million |
Nurix Therapeutics | Heterobifunctional Degraders | NX-2127; NX-5948 | Underwritten Public Offering | Apr-24 | NX-2127: Phase Ib, NX-5948: Fast-track designation | $175 million |
Kymera Therapeutics | Heterobifunctional Degraders | KT-474 | Underwritten Public Offering | Jan-24 | Phase IIb | $275 million |
Magnet Biomedicine | Molecular Glues | TrueGlues™ | Series A | Sep-23 | Discovery | $50 million |
Cullgen | TPD | uSMITE™ | Series C | May-23 | Preclinical | $35 million |
Anhorn Medicines | Bi-functional degraders & Molecular Glues | Undisclosed | Series A | Apr-23 | Discovery | $10 million |
Degron Therapeutics | Molecular Glues | GlueXplorer® platform | Series A | Jun-22 | Discovery | $22 million |
Triana Biomedicine | Molecular Glues | Undisclosed | Series A | Apr-22 | Discovery | $110 million |
Plexium | Molecular Glues | Undisclosed | Oversubscribed Financing Round | Feb-22 | Preclinical | $102 million |
Ambagon Therapeutics | Molecular Glues | Undisclosed | Series A | Jan-22 | Discovery | $85 Million |
Ranok Therapeutics | TPD | CHAMP™ | Series B | Aug-21 | Discovery | $40 Million |
Orum Therapeutics | TPD | AnDC™ | Series B | Jun-21 | Preclinical | $84 Million |
Monte Rosa Therapeutics | Molecular Glues | Undisclosed | Series C | Mar-21 | Preclinical | $95 million |
Cullgen | TPD | Undisclosed | Series B | Feb-21 | Preclinical | $50 million |
Neomorph | Molecular Glues | Undisclosed | Series A | Dec-20 | N/A | $109 million |
Monte Rosa Therapeutics | Molecular Glues | Undisclosed | Series B | Sep-20 | N/A | $96 million |
C4 Therapeutics | Molecular Glues | TORPEDO™ platform | Series B | Jun-20 | Preclinical | $170 million |
Cullgen | TPD | Undisclosed | Series A | Apr-19 | Preclinical | $16 million |
Cullgen | TPD | Undisclosed | Series Seed | Mar-18 | N/A | $15 million |
Table 4. Comparison list of TPDs 26
Table 5. Recent list of Targeted Protein Degradation Molecules
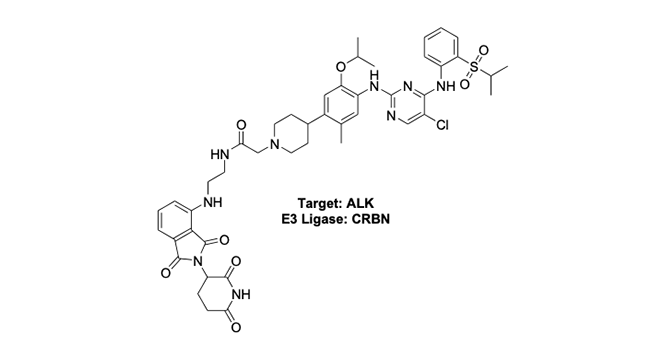
Zhang, C.; Han, X.-R.; Yang, X.; Jiang, B.; Liu, J.; Xiong, Y.; Jin, J. Proteolysis Targeting Chimeras (PROTACs) of Anaplastic Lymphoma Kinase (ALK). Eur. J. Med. Chem. 2018, 151, 304–314. https://doi.org/10.1016/j.ejmech.2018.03.071.
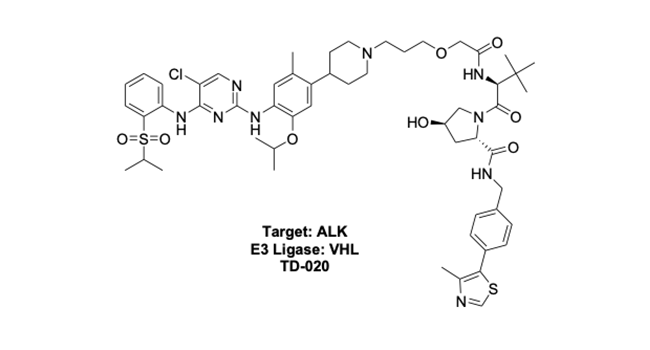
Kang, C. H.; Lee, D. H.; Lee, C. O.; Du Ha, J.; Park, C. H.; Hwang, J. Y. Induced Protein Degradation of Anaplastic Lymphoma Kinase (ALK) by Proteolysis Targeting Chimera (PROTAC). Biochem. Biophys. Res. Commun. 2018, 505 (2), 542–547. https://doi.org/10.1016/j.bbrc.2018.09.169.
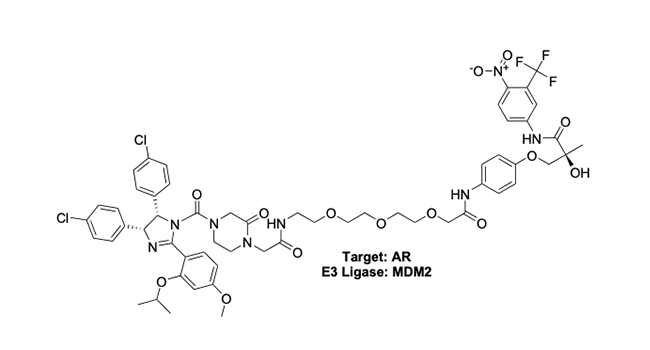
Schneekloth, A. R.; Pucheault, M.; Tae, H. S.; Crews, C. M. Targeted Intracellular Protein Degradation Induced by a Small Molecule: En Route to Chemical Proteomics. Bioorg. Med. Chem. Lett. 2008, 18 (22), 5904–5908. https://doi.org/10.1016/j.bmcl.2008.07.114.
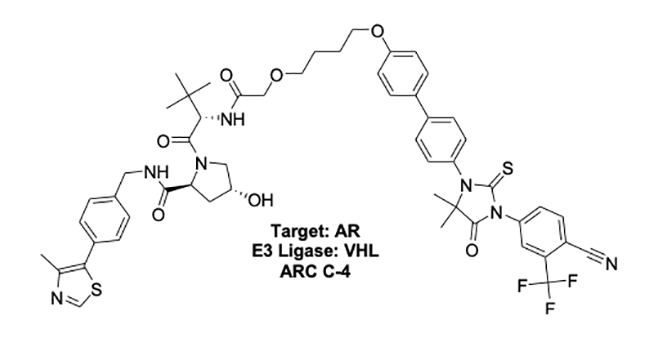
Salami, J.; Alabi, S.; Willard, R. R.; Vitale, N. J.; Wang, J.; Dong, H.; Jin, M.; McDonnell, D. P.; Crew, A. P.; Neklesa, T. K.; Crews, C. M. Androgen Receptor Degradation by the Proteolysis-Targeting Chimera ARCC-4 Outperforms Enzalutamide in Cellular Models of Prostate Cancer Drug Resistance. Commun. Biol. 2018, 1 (1), 100. https://doi.org/10.1038/s42003-018-0105-8.
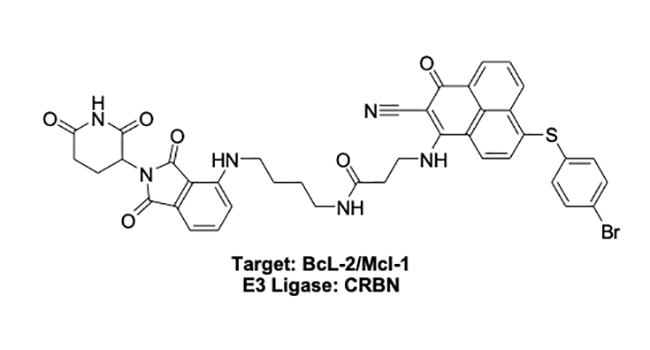
Wang, Z.; He, N.; Guo, Z.; Niu, C.; Song, T.; Guo, Y.; Cao, K.; Wang, A.; Zhu, J.; Zhang, X.; Zhang, Z. Proteolysis Targeting Chimeras for the Selective Degradation of Mcl-1/Bcl-2 Derived from Nonselective Target Binding Ligands. J. Med. Chem. 2019, 62 (17), 8152–8163. https://doi.org/10.1021/acs.jmedchem.9b00919.
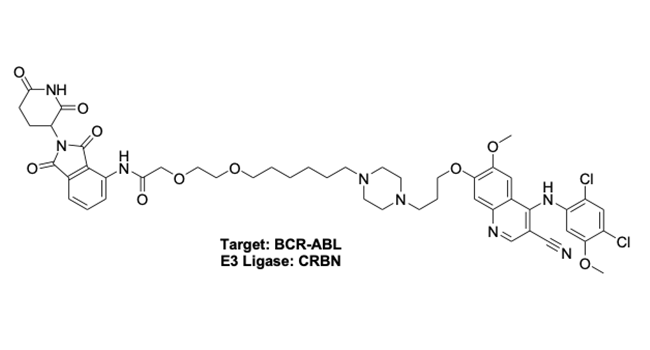
Lai, A. C.; Toure, M.; Hellerschmied, D.; Salami, J.; Jaime-Figueroa, S.; Ko, E.; Hines, J.; Crews, C. M. Modular PROTAC Design for the Degradation of Oncogenic BCR-ABL. Angew. Chemie Int. Ed. 2016, 55 (2), 807–810. https://doi.org/10.1002/anie.201507634.
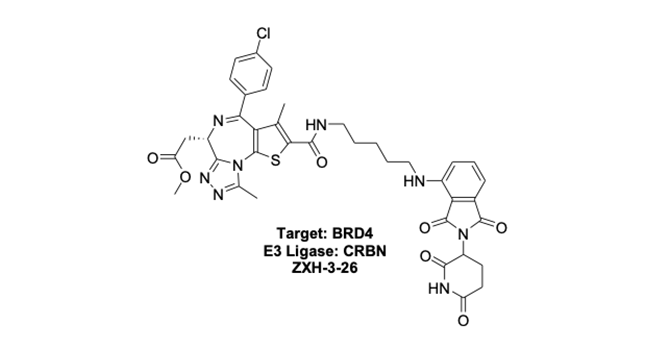
Nowak, R. P.; DeAngelo, S. L.; Buckley, D.; He, Z.; Donovan, K. A.; An, J.; Safaee, N.; Jedrychowski, M. P.; Ponthier, C. M.; Ishoey, M.; Zhang, T.; Mancias, J. D.; Gray, N. S.; Bradner, J. E.; Fischer, E. S. Plasticity in Binding Confers Selectivity in Ligand-Induced Protein Degradation. Nat. Chem. Biol. 2018, 14 (7), 706–714. https://doi.org/10.1038/s41589-018-0055-y.
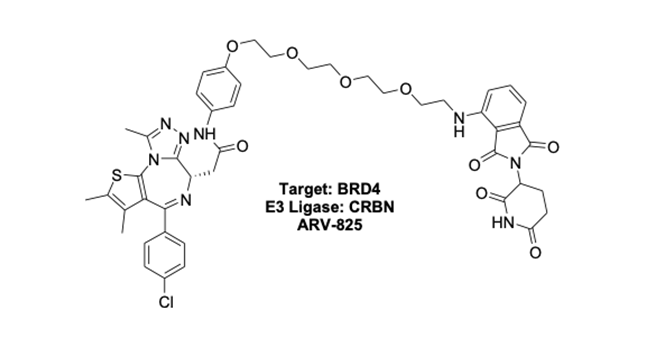
Lu, J.; Qian, Y.; Altieri, M.; Dong, H.; Wang, J.; Raina, K.; Hines, J.; Winkler, J. D.; Crew, A. P.; Coleman, K.; Crews, C. M. Hijacking the E3 Ubiquitin Ligase Cereblon to Efficiently Target BRD4. Chem. Biol. 2015, 22 (6), 755–763. https://doi.org/10.1016/j.chembiol.2015.05.009.
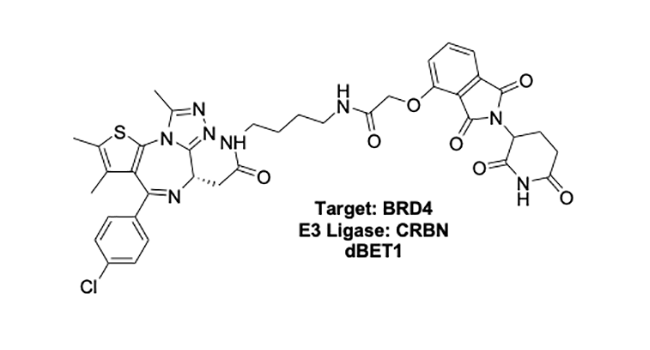
Winter, G. E.; Buckley, D. L.; Paulk, J.; Roberts, J. M.; Souza, A.; Dhe-Paganon, S.; Bradner, J. E. Phthalimide Conjugation as a Strategy for in Vivo Target Protein Degradation. Science 2015, 348 (6241), 1376–1381. https://doi.org/10.1126/science.aab1433.
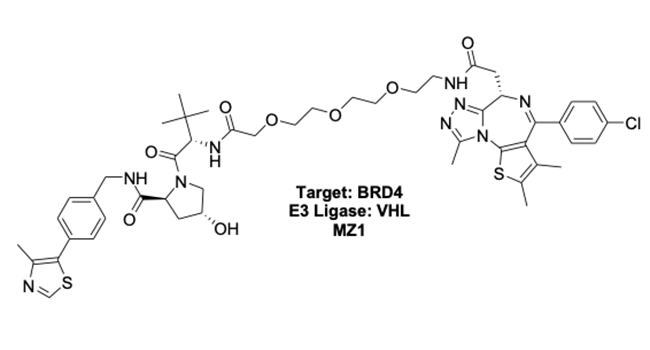
Zengerle, M.; Chan, K.-H.; Ciulli, A. Selective Small Molecule Induced Degradation of the BET Bromodomain Protein BRD4. ACS Chem. Biol. 2015, 10 (8), 1770–1777. https://doi.org/10.1021/acschembio.5b00216.
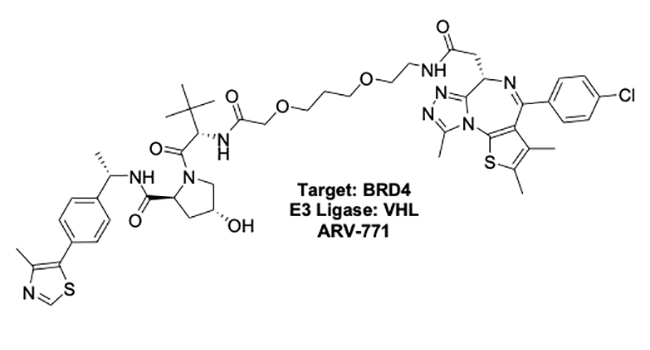
Raina, K.; Lu, J.; Qian, Y.; Altieri, M.; Gordon, D.; Rossi, A. M. K.; Wang, J.; Chen, X.; Dong, H.; Siu, K.; Winkler, J. D.; Crew, A. P.; Crews, C. M.; Coleman, K. G. PROTAC-Induced BET Protein Degradation as a Therapy for Castration-Resistant Prostate Cancer. Proc. Natl. Acad. Sci. 2016, 113 (26), 7124–7129. https://doi.org/10.1073/pnas.1521738113.
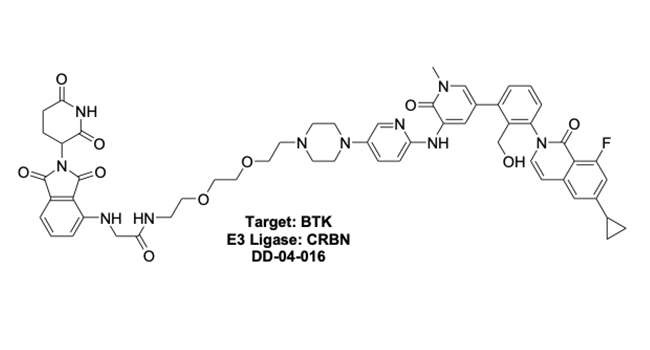
Huang, H.-T.; Dobrovolsky, D.; Paulk, J.; Yang, G.; Weisberg, E. L.; Doctor, Z. M.; Buckley, D. L.; Cho, J.-H.; Ko, E.; Jang, J.; Shi, K.; Choi, H. G.; Griffin, J. D.; Li, Y.; Treon, S. P.; Fischer, E. S.; Bradner, J. E.; Tan, L.; Gray, N. S. A Chemoproteomic Approach to Query the Degradable Kinome Using a Multi-Kinase Degrader. Cell Chem. Biol. 2018, 25 (1), 88-99.e6. https://doi.org/10.1016/j.chembiol.2017.10.005.
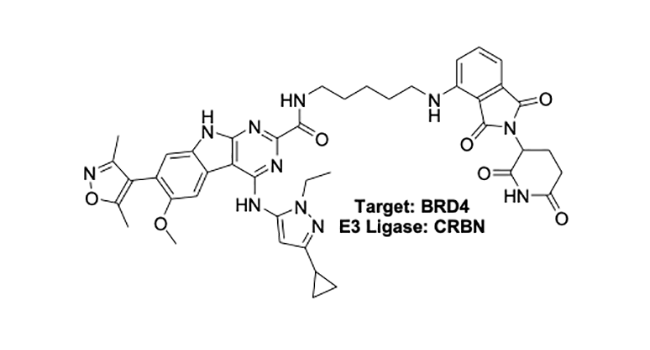
Zhou, B.; Hu, J.; Xu, F.; Chen, Z.; Bai, L.; Fernandez-Salas, E.; Lin, M.; Liu, L.; Yang, C.-Y.; Zhao, Y.; McEachern, D.; Przybranowski, S.; Wen, B.; Sun, D.; Wang, S. Discovery of a Small-Molecule Degrader of Bromodomain and Extra-Terminal (BET) Proteins with Picomolar Cellular Potencies and Capable of Achieving Tumor Regression. J. Med. Chem. 2018, 61 (2), 462–481. https://doi.org/10.1021/acs.jmedchem.6b01816.
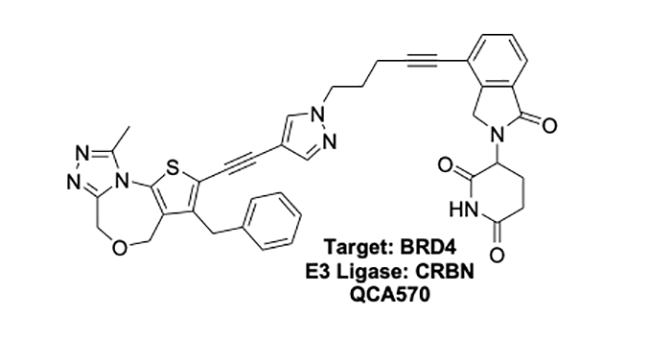
Qin, C.; Hu, Y.; Zhou, B.; Fernandez-Salas, E.; Yang, C.-Y.; Liu, L.; McEachern, D.; Przybranowski, S.; Wang, M.; Stuckey, J.; Meagher, J.; Bai, L.; Chen, Z.; Lin, M.; Yang, J.; Ziazadeh, D. N.; Xu, F.; Hu, J.; Xiang, W.; Huang, L.; Li, S.; Wen, B.; Sun, D.; Wang, S. Discovery of QCA570 as an Exceptionally Potent and Efficacious Proteolysis Targeting Chimera (PROTAC) Degrader of the Bromodomain and Extra-Terminal (BET) Proteins Capable of Inducing Complete and Durable Tumor Regression. J. Med. Chem. 2018, 61 (15), 6685–6704. https://doi.org/10.1021/acs.jmedchem.8b00506.
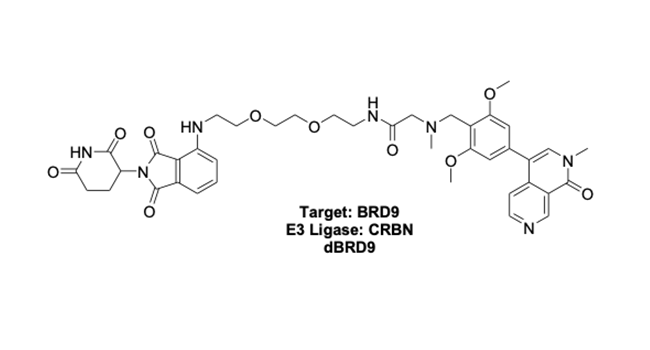
Remillard, D.; Buckley, D. L.; Paulk, J.; Brien, G. L.; Sonnett, M.; Seo, H.-S.; Dastjerdi, S.; Wühr, M.; Dhe-Paganon, S.; Armstrong, S. A.; Bradner, J. E. Degradation of the BAF Complex Factor BRD9 by Heterobifunctional Ligands. Angew. Chemie Int. Ed. 2017, 56 (21), 5738–5743. https://doi.org/10.1002/anie.201611281.
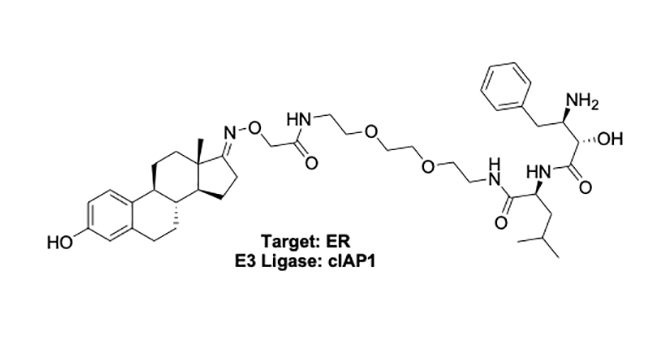
Itoh, Y.; Kitaguchi, R.; Ishikawa, M.; Naito, M.; Hashimoto, Y. Design, Synthesis and Biological Evaluation of Nuclear Receptor-Degradation Inducers. Bioorg. Med. Chem. 2011, 19 (22), 6768–6778. https://doi.org/10.1016/j.bmc.2011.09.041.
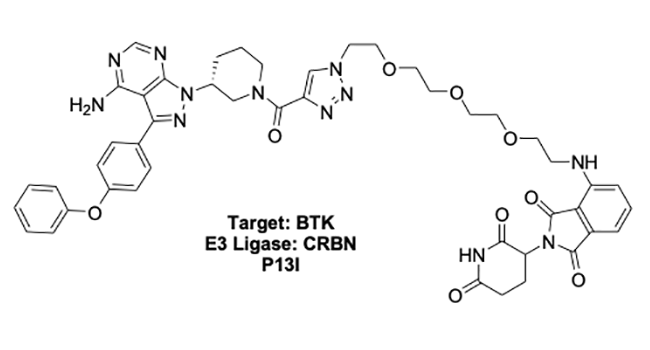
Sun, Y.; Zhao, X.; Ding, N.; Gao, H.; Wu, Y.; Yang, Y.; Zhao, M.; Hwang, J.; Song, Y.; Liu, W.; Rao, Y. PROTAC-Induced BTK Degradation as a Novel Therapy for Mutated BTK C481S Induced Ibrutinib-Resistant B-Cell Malignancies. Cell Res. 2018, 28 (7), 779–781. https://doi.org/10.1038/s41422-018-0055-1.
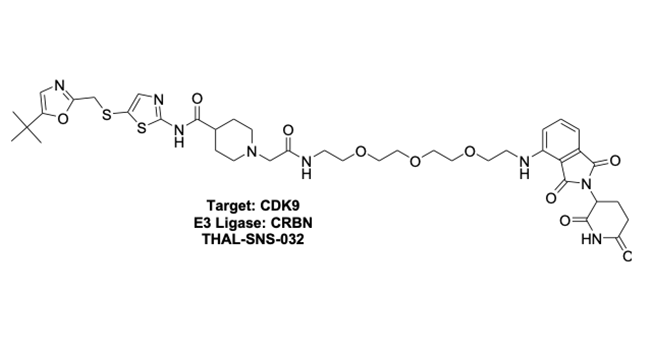
Olson, C. M.; Jiang, B.; Erb, M. A.; Liang, Y.; Doctor, Z. M.; Zhang, Z.; Zhang, T.; Kwiatkowski, N.; Boukhali, M.; Green, J. L.; Haas, W.; Nomanbhoy, T.; Fischer, E. S.; Young, R. A.; Bradner, J. E.; Winter, G. E.; Gray, N. S. Pharmacological Perturbation of CDK9 Using Selective CDK9 Inhibition or Degradation. Nat. Chem. Biol. 2018, 14 (2), 163–170. https://doi.org/10.1038/nchembio.2538.
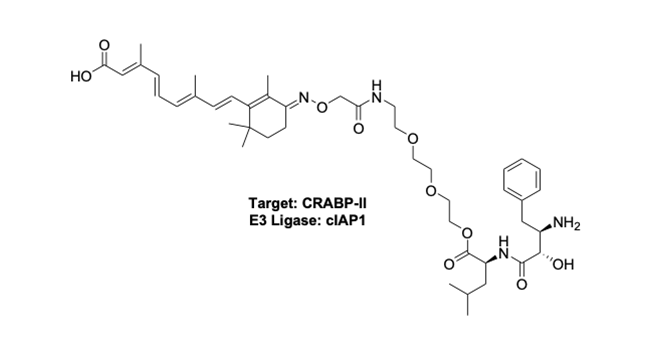
Itoh, Y.; Ishikawa, M.; Naito, M.; Hashimoto, Y. Protein Knockdown Using Methyl Bestatin−Ligand Hybrid Molecules: Design and Synthesis of Inducers of Ubiquitination-Mediated Degradation of Cellular Retinoic Acid-Binding Proteins. J. Am. Chem. Soc. 2010, 132 (16), 5820–5826. https://doi.org/10.1021/ja100691p.
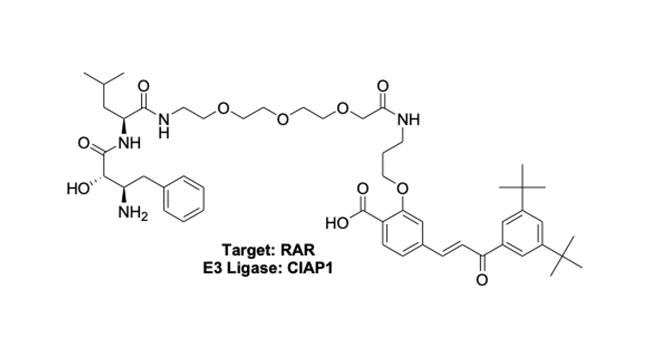
Itoh, Y.; Kitaguchi, R.; Ishikawa, M.; Naito, M.; Hashimoto, Y. Design, Synthesis and Biological Evaluation of Nuclear Receptor-Degradation Inducers. Bioorg. Med. Chem. 2011, 19 (22), 6768–6778. https://doi.org/10.1016/j.bmc.2011.09.041.
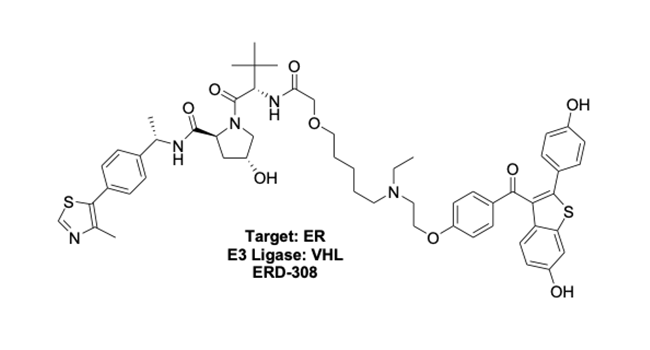
Hu, J.; Hu, B.; Wang, M.; Xu, F.; Miao, B.; Yang, C.-Y.; Wang, M.; Liu, Z.; Hayes, D. F.; Chinnaswamy, K.; Delproposto, J.; Stuckey, J.; Wang, S. Discovery of ERD-308 as a Highly Potent Proteolysis Targeting Chimera (PROTAC) Degrader of Estrogen Receptor (ER). J. Med. Chem. 2019, 62 (3), 1420–1442. https://doi.org/10.1021/acs.jmedchem.8b01572.
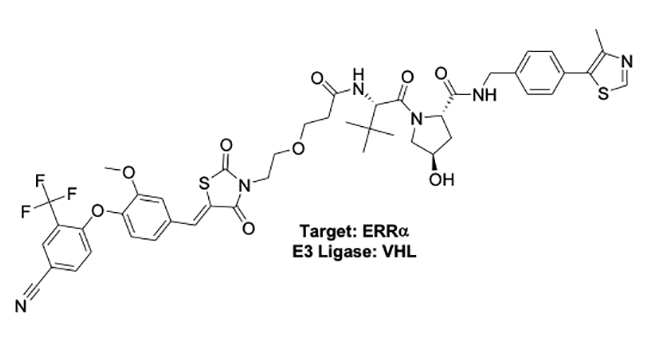
Bondeson, D. P.; Mares, A.; Smith, I. E. D.; Ko, E.; Campos, S.; Miah, A. H.; Mulholland, K. E.; Routly, N.; Buckley, D. L.; Gustafson, J. L.; Zinn, N.; Grandi, P.; Shimamura, S.; Bergamini, G.; Faelth-Savitski, M.; Bantscheff, M.; Cox, C.; Gordon, D. A.; Willard, R. R.; Flanagan, J. J.; Casillas, L. N.; Votta, B. J.; den Besten, W.; Famm, K.; Kruidenier, L.; Carter, P. S.; Harling, J. D.; Churcher, I.; Crews, C. M. Catalytic in Vivo Protein Knockdown by Small-Molecule PROTACs. Nat. Chem. Biol. 2015, 11 (8), 611–617. https://doi.org/10.1038/nchembio.1858.
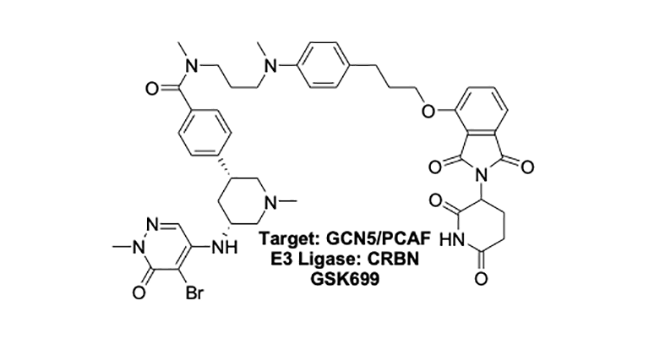
Bassi, Z. I.; Fillmore, M. C.; Miah, A. H.; Chapman, T. D.; Maller, C.; Roberts, E. J.; Davis, L. C.; Lewis, D. E.; Galwey, N. W.; Waddington, K. E.; Parravicini, V.; Macmillan-Jones, A. L.; Gongora, C.; Humphreys, P. G.; Churcher, I.; Prinjha, R. K.; Tough, D. F. Modulating PCAF/GCN5 Immune Cell Function through a PROTAC Approach. ACS Chem. Biol. 2018, 13 (10), 2862–2867. https://doi.org/10.1021/acschembio.8b00705.
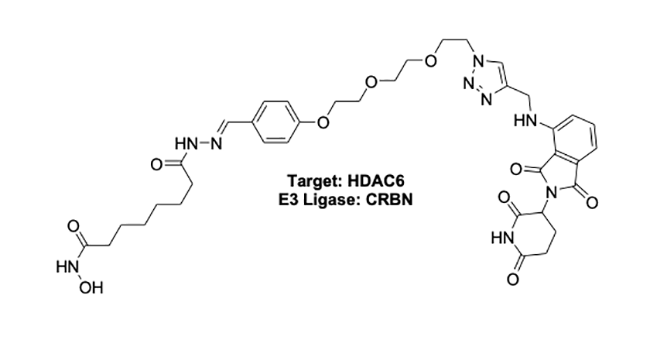
Yang, K.; Song, Y.; Xie, H.; Wu, H.; Wu, Y.-T.; Leisten, E. D.; Tang, W. Development of the First Small Molecule Histone Deacetylase 6 (HDAC6) Degraders. Bioorg. Med. Chem. Lett. 2018, 28 (14), 2493–2497. https://doi.org/10.1016/j.bmcl.2018.05.057.
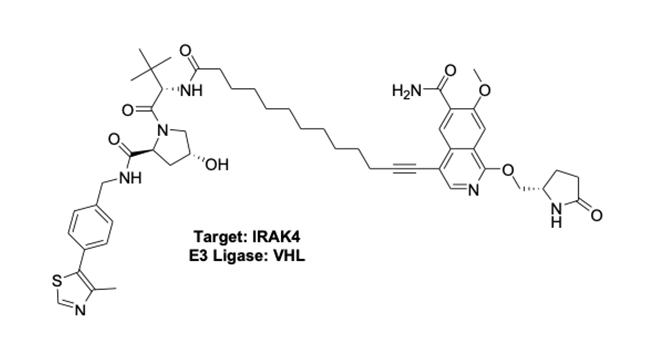
Nunes, J.; McGonagle, G. A.; Eden, J.; Kiritharan, G.; Touzet, M.; Lewell, X.; Emery, J.; Eidam, H.; Harling, J. D.; Anderson, N. A. Targeting IRAK4 for Degradation with PROTACs. ACS Med. Chem. Lett. 2019, 10 (7), 1081–1085. https://doi.org/10.1021/acsmedchemlett.9b00219.

Li, W.; Gao, C.; Zhao, L.; Yuan, Z.; Chen, Y.; Jiang, Y. Phthalimide Conjugations for the Degradation of Oncogenic PI3K. Eur. J. Med. Chem. 2018, 151, 237–247. https://doi.org/10.1016/j.ejmech.2018.03.066.
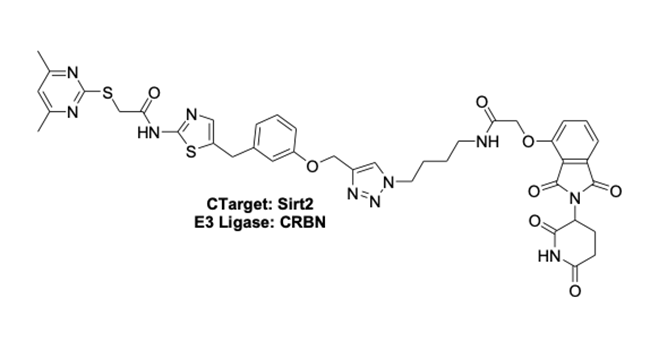
Schiedel, M.; Herp, D.; Hammelmann, S.; Swyter, S.; Lehotzky, A.; Robaa, D.; Oláh, J.; Ovádi, J.; Sippl, W.; Jung, M. Chemically Induced Degradation of Sirtuin 2 (Sirt2) by a Proteolysis Targeting Chimera (PROTAC) Based on Sirtuin Rearranging Ligands (SirReals). J. Med. Chem. 2018, 61 (2), 482–491. https://doi.org/10.1021/acs.jmedchem.6b01872.
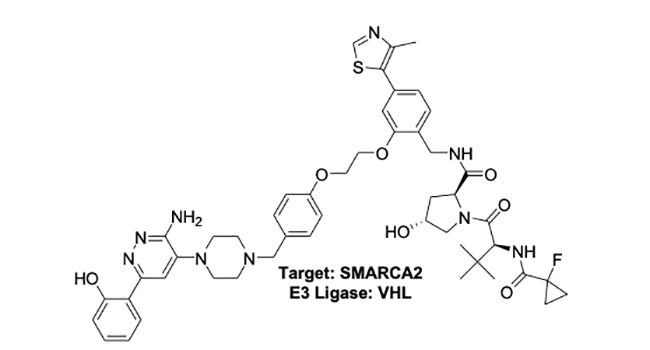
Farnaby, W.; Koegl, M.; Roy, M. J.; Whitworth, C.; Diers, E.; Trainor, N.; Zollman, D.; Steurer, S.; Karolyi-Oezguer, J.; Riedmueller, C.; Gmaschitz, T.; Wachter, J.; Dank, C.; Galant, M.; Sharps, B.; Rumpel, K.; Traxler, E.; Gerstberger, T.; Schnitzer, R.; Petermann, O.; Greb, P.; Weinstabl, H.; Bader, G.; Zoephel, A.; Weiss-Puxbaum, A.; Ehrenhöfer-Wölfer, K.; Wöhrle, S.; Boehmelt, G.; Rinnenthal, J.; Arnhof, H.; Wiechens, N.; Wu, M.-Y.; Owen-Hughes, T.; Ettmayer, P.; Pearson, M.; McConnell, D. B.; Ciulli, A. BAF Complex Vulnerabilities in Cancer Demonstrated via Structure-Based PROTAC Design. Nat. Chem. Biol. 2019, 15 (7), 672–680. https://doi.org/10.1038/s41589-019-0294-6.
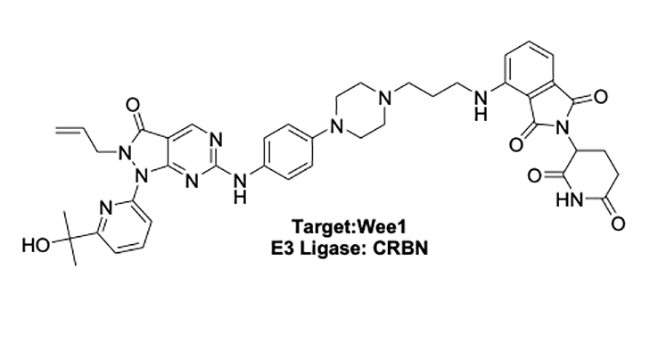
Li, Z.; Pinch, B. J.; Olson, C. M.; Donovan, K. A.; Nowak, R. P.; Mills, C. E.; Scott, D. A.; Doctor, Z. M.; Eleuteri, N. A.; Chung, M.; Sorger, P. K.; Fischer, E. S.; Gray, N. S. Development and Characterization of a Wee1 Kinase Degrader. Cell Chem. Biol. 2020, 27 (1), 57-65.e9. https://doi.org/10.1016/j.chembiol.2019.10.013.
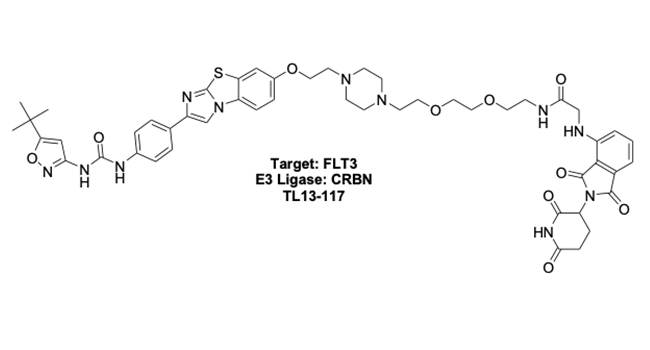
Huang, H.-T.; Dobrovolsky, D.; Paulk, J.; Yang, G.; Weisberg, E. L.; Doctor, Z. M.; Buckley, D. L.; Cho, J.-H.; Ko, E.; Jang, J.; Shi, K.; Choi, H. G.; Griffin, J. D.; Li, Y.; Treon, S. P.; Fischer, E. S.; Bradner, J. E.; Tan, L.; Gray, N. S. A Chemoproteomic Approach to Query the Degradable Kinome Using a Multi-Kinase Degrader. Cell Chem. Biol. 2018, 25 (1), 88-99.e6. https://doi.org/10.1016/j.chembiol.2017.10.005.
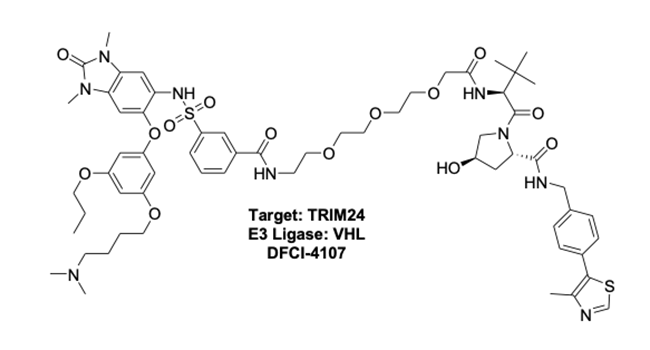
Gechijian, L. N.; Buckley, D. L.; Lawlor, M. A.; Reyes, J. M.; Paulk, J.; Ott, C. J.; Winter, G. E.; Erb, M. A.; Scott, T. G.; Xu, M.; Seo, H.-S.; Dhe-Paganon, S.; Kwiatkowski, N. P.; Perry, J. A.; Qi, J.; Gray, N. S.; Bradner, J. E. Functional TRIM24 Degrader via Conjugation of Ineffectual Bromodomain and VHL Ligands. Nat. Chem. Biol. 2018, 14 (4), 405–412. https://doi.org/10.1038/s41589-018-0010-y.
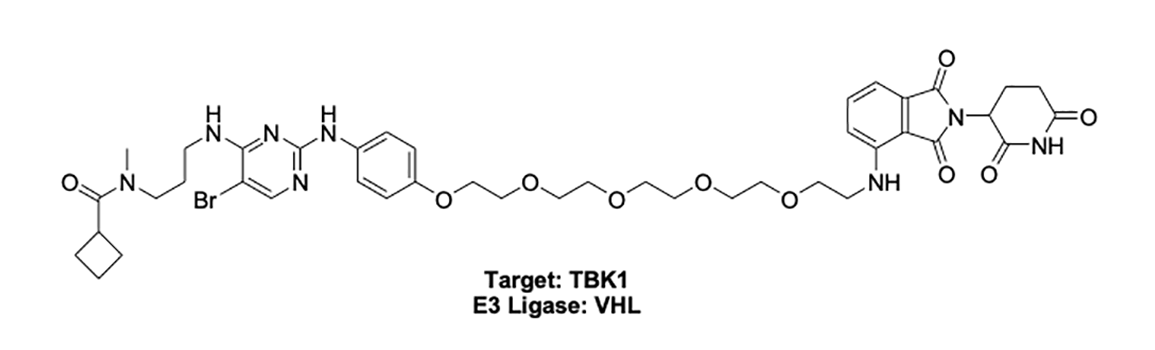
Crew, A. P.; Raina, K.; Dong, H.; Qian, Y.; Wang, J.; Vigil, D.; Serebrenik, Y. V.; Hamman, B. D.; Morgan, A.; Ferraro, C.; Siu, K.; Neklesa, T. K.; Winkler, J. D.; Coleman, K. G.; Crews, C. M. Identification and Characterization of Von Hippel-Lindau-Recruiting Proteolysis Targeting Chimeras (PROTACs) of TANK-Binding Kinase 1. J. Med. Chem. 2018, 61 (2), 583–598. https://doi.org/10.1021/acs.jmedchem.7b00635.
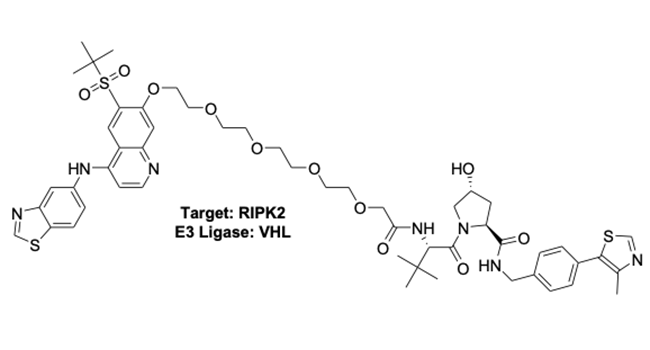
Bondeson, D. P.; Mares, A.; Smith, I. E. D.; Ko, E.; Campos, S.; Miah, A. H.; Mulholland, K. E.; Routly, N.; Buckley, D. L.; Gustafson, J. L.; Zinn, N.; Grandi, P.; Shimamura, S.; Bergamini, G.; Faelth-Savitski, M.; Bantscheff, M.; Cox, C.; Gordon, D. A.; Willard, R. R.; Flanagan, J. J.; Casillas, L. N.; Votta, B. J.; den Besten, W.; Famm, K.; Kruidenier, L.; Carter, P. S.; Harling, J. D.; Churcher, I.; Crews, C. M. Catalytic in Vivo Protein Knockdown by Small-Molecule PROTACs. Nat. Chem. Biol. 2015, 11 (8), 611–617. https://doi.org/10.1038/nchembio.1858.
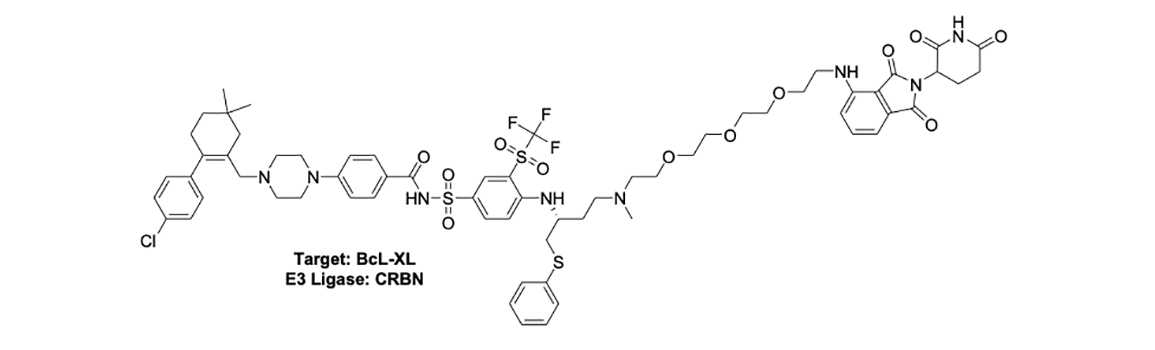
Zhang, X.; Thummuri, D.; Liu, X.; Hu, W.; Zhang, P.; Khan, S.; Yuan, Y.; Zhou, D.; Zheng, G. Discovery of PROTAC BCL-XL Degraders as Potent Anticancer Agents with Low on-Target Platelet Toxicity. Eur. J. Med. Chem. 2020, 192, 112186. https://doi.org/10.1016/j.ejmech.2020.112186.
References
Review Articles for Targeted Protein Degradation
- Lai, A. C.; Crews, C. M. Induced Protein Degradation: An Emerging Drug Discovery Paradigm. Nature Reviews Drug Discovery 2017, 16 (2), 101–114. https://doi.org/10.1038/nrd.2016.211.
- Zou, Y.; Ma, D.; Wang, Y. The PROTAC Technology in Drug Development. Cell Biochemistry and Function 2019, 37 (1), 21–30. https://doi.org/10.1002/cbf.3369.
- Wang, Y.; Jiang, X.; Feng, F.; Liu, W.; Sun, H. Degradation of Proteins by PROTACs and Other Strategies. Acta Pharmaceutica Sinica B 2020, 10 (2), 207–238. https://doi.org/10.1016/j.apsb.2019.08.001.
- An, S.; Fu, L. Small-Molecule PROTACs: An Emerging and Promising Approach for the Development of Targeted Therapy Drugs. EBioMedicine 2018, 36, 553–562. https://doi.org/10.1016/j.ebiom.2018.09.005.
- Cromm, P. M.; Crews, C. M. Targeted Protein Degradation: From Chemical Biology to Drug Discovery. Cell Chemical Biology 2017, 24 (9), 1181–1190. https://doi.org/10.1016/j.chembiol.2017.05.024.
Specific References
(1) Hanna, J.; Guerra-Moreno, A.; Ang, J.; Micoogullari, Y. Protein Degradation and the Pathologic Basis of Disease. American Journal of Pathology. Elsevier Inc. January 1, 2019, pp 94–103. https://doi.org/10.1016/j.ajpath.2018.09.004.
(2) Kramer, L. T.; Zhang, X. Expanding the Landscape of E3 Ligases for Targeted Protein Degradation. Current Research in Chemical Biology 2022, 2, 100020. https://doi.org/10.1016/j.crchbi.2022.100020.
(3) Békés, M.; Langley, D. R.; Crews, C. M. PROTAC Targeted Protein Degraders: The Past Is Prologue. Nature Reviews Drug Discovery. Nature Research March 1, 2022, pp 181–200. https://doi.org/10.1038/s41573-021-00371-6.
(4) Lai, A. C.; Crews, C. M. Induced Protein Degradation: An Emerging Drug Discovery Paradigm. Nature Reviews Drug Discovery. Nature Publishing Group February 2, 2017, pp 101–114. https://doi.org/10.1038/nrd.2016.211.
(5) Zou, Y.; Ma, D.; Wang, Y. The PROTAC Technology in Drug Development. Cell Biochemistry and Function. John Wiley and Sons Ltd January 1, 2019, pp 21–30. https://doi.org/10.1002/cbf.3369.
(6) Wang, Y.; Jiang, X.; Feng, F.; Liu, W.; Sun, H. Degradation of Proteins by PROTACs and Other Strategies. Acta Pharmaceutica Sinica B. Chinese Academy of Medical Sciences February 1, 2020, pp 207–238. https://doi.org/10.1016/j.apsb.2019.08.001.
(7) An, S.; Fu, L. Small-Molecule PROTACs: An Emerging and Promising Approach for the Development of Targeted Therapy Drugs. EBioMedicine. Elsevier B.V. October 1, 2018, pp 553–562. https://doi.org/10.1016/j.ebiom.2018.09.005.
(8) Cromm, P. M.; Crews, C. M. Targeted Protein Degradation: From Chemical Biology to Drug Discovery. Cell Chemical Biology. Elsevier Ltd September 21, 2017, pp 1181–1190. https://doi.org/10.1016/j.chembiol.2017.05.024.
(9) Powell, M.; Blaskovich, M. A. T.; Hansford, K. A. Targeted Protein Degradation: The New Frontier of Antimicrobial Discovery? ACS Infectious Diseases. American Chemical Society August 13, 2021, pp 2050–2067. https://doi.org/10.1021/acsinfecdis.1c00203.
(10) Békés, M.; Langley, D. R.; Crews, C. M. PROTAC Targeted Protein Degraders: The Past Is Prologue. Nature Reviews Drug Discovery. Nature Research March 1, 2022, pp 181–200. https://doi.org/10.1038/s41573-021-00371-6.
(11) Faust, T. B.; Donovan, K. A.; Yue, H.; Chamberlain, P. P.; Fischer, E. S. Small-Molecule Approaches to Targeted Protein Degradation. Annu. Rev. Cancer Biol. 2021 2021, 5, 181–201. https://doi.org/10.1146/annurev-cancerbio-051420.
(12) Arthur, R.; Valle-Argos, B.; Steele, A. J.; Packham, G. Development of PROTACs to Address Clinical Limitations Associated with BTK-Targeted Kinase Inhibitors. Exploration of Targeted Anti-tumor Therapy. Open Exploration Publishing Inc 2020, pp 131–152. https://doi.org/10.37349/etat.2020.00009.
(13) Salami, J.; Crews, C. M. Waste Disposal-An Attractive Strategy for Cancer Therapy. https://doi.org/10.1126/science.aam7340
(14) Li, H.; Dong, J.; Cai, M.; Xu, Z.; Cheng, X. D.; Qin, J. J. Protein Degradation Technology: A Strategic Paradigm Shift in Drug Discovery. Journal of Hematology and Oncology. BioMed Central Ltd December 1, 2021. https://doi.org/10.1186/s13045-021-01146-7.
(15) Bondeson, D. P.; Smith, B. E.; Burslem, G. M.; Buhimschi, A. D.; Hines, J.; Jaime-Figueroa, S.; Wang, J.; Hamman, B. D.; Ishchenko, A.; Crews, C. M. Lessons in PROTAC Design from Selective Degradation with a Promiscuous Warhead. Cell Chem Biol 2018, 25 (1), 78-87.e5. https://doi.org/10.1016/j.chembiol.2017.09.010.
(16) Naito, M.; Ohoka, N.; Shibata, N. SNIPERs—Hijacking IAP Activity to Induce Protein Degradation. Drug Discovery Today: Technologies. Elsevier Ltd April 1, 2019, pp 35–42. https://doi.org/10.1016/j.ddtec.2018.12.002.
(17) Hughes, S. J.; Ciulli, A. Molecular Recognition of Ternary Complexes: A New Dimension in the Structure-Guided Design of Chemical Degraders. Essays in Biochemistry. Portland Press Ltd November 8, 2017, pp 505–516. https://doi.org/10.1042/EBC20170041.
(18) Takahashi, D.; Moriyama, J.; Nakamura, T.; Miki, E.; Takahashi, E.; Sato, A.; Akaike, T.; Itto-Nakama, K.; Arimoto, H. AUTACs: Cargo-Specific Degraders Using Selective Autophagy. Mol Cell 2019, 76 (5), 797-810.e10. https://doi.org/10.1016/j.molcel.2019.09.009.
(19) Banik, S. M.; Pedram, K.; Wisnovsky, S.; Ahn, G.; Riley, N. M.; Bertozzi, C. R. Lysosome-Targeting Chimaeras for Degradation of Extracellular Proteins. Nature 2020, 584 (7820), 291–297. https://doi.org/10.1038/s41586-020-2545-9.
(20) Costales, M. G.; Matsumoto, Y.; Velagapudi, S. P.; Disney, M. D. Small Molecule Targeted Recruitment of a Nuclease to RNA. J Am Chem Soc 2018, 140 (22), 6741–6744. https://doi.org/10.1021/jacs.8b01233.
(21) Yamazoe, S.; Tom, J.; Fu, Y.; Wu, W.; Zeng, L.; Sun, C.; Liu, Q.; Lin, J.; Lin, K.; Fairbrother, W. J.; Staben, S. T. Heterobifunctional Molecules Induce Dephosphorylation of Kinases-A Proof of Concept Study. J Med Chem 2020, 63 (6), 2807–2813. https://doi.org/10.1021/acs.jmedchem.9b01167.
(22) Lebraud, H.; Wright, D. J.; Johnson, C. N.; Heightman, T. D. Protein Degradation by In-Cell Self-Assembly of Proteolysis Targeting Chimeras. ACS Cent Sci 2016, 2 (12), 927–934. https://doi.org/10.1021/acscentsci.6b00280.
(23) Zhao, L.; Zhao, J.; Zhong, K.; Tong, A.; Jia, D. Targeted Protein Degradation: Mechanisms, Strategies and Application. Signal Transduction and Targeted Therapy. Springer Nature December 1, 2022. https://doi.org/10.1038/s41392-022-00966-4.
(24) Sakamoto, K. M.; Kim, K. B.; Kumagai, A.; Mercurio, F.; Crews, C. M.; Deshaies, R. J. Protacs: Chimeric Molecules That Target Proteins to the Skp1-Cullin-F Box Complex for Ubiquitination and Degradation; 2001. https://doi.org/10.1073/pnas.141230798
(25) Mullard, A. Targeted Protein Degraders Crowd into the Clinic. Nature reviews. Drug discovery. NLM (Medline) April 1, 2021, pp 247–250. https://doi.org/10.1038/d41573-021-00052-4.
(26) He, M.; Cao, C.; Ni, Z.; Liu, Y.; Song, P.; Hao, S.; He, Y.; Sun, X.; Rao, Y. PROTACs: Great Opportunities for Academia and Industry (an Update from 2020 to 2021). Signal Transduction and Targeted Therapy. Springer Nature December 1, 2022. https://doi.org/10.1038/s41392-022-00999-9.
(27) Neklesa, T. K.; Snyder, L. B.; Willard, R. R.; Vitale, N.; Pizzano, J.; Gordon, D. A.; Bookbinder, M.; Macaluso, J.; Dong, H.; Ferraro, C.; Wang, G.; Wang, J.; Crews, C. M.; Houston, J.; Crew, A. P.; Taylor, I. ARV-110: An Oral Androgen Receptor PROTAC Degrader for Prostate Cancer. https://doi.org/10.1200/JCO.2019.37.7_suppl.259
(28) Lin, X.; Xiang, H.; Luo, G. Targeting Estrogen Receptor α for Degradation with PROTACs: A Promising Approach to Overcome Endocrine Resistance. European Journal of Medicinal Chemistry. Elsevier Masson s.r.l. November 15, 2020. https://doi.org/10.1016/j.ejmech.2020.112689.
(29) Brown, D. G.; Boström, J. Where Do Recent Small Molecule Clinical Development Candidates Come From? J Med Chem 2018, 61 (21), 9442–9468. https://doi.org/10.1021/acs.jmedchem.8b00675.
(30) Schapira, M.; Calabrese, M. F.; Bullock, A. N.; Crews, C. M. Targeted Protein Degradation: Expanding the Toolbox. Nat Rev Drug Discov 2019, 18 (12), 949–963. https://doi.org/10.1038/s41573-019-0047-y.
(31) Blaquiere, N.; Villemure, E.; Staben, S. T. Medicinal Chemistry of Inhibiting RING-Type E3 Ubiquitin Ligases. Journal of Medicinal Chemistry. American Chemical Society August 13, 2020, pp 7957–7985. https://doi.org/10.1021/acs.jmedchem.9b01451.
(32) Gadd, M. S.; Testa, A.; Lucas, X.; Chan, K. H.; Chen, W.; Lamont, D. J.; Zengerle, M.; Ciulli, A. Structural Basis of PROTAC Cooperative Recognition for Selective Protein Degradation. Nat Chem Biol 2017, 13 (5), 514–521. https://doi.org/10.1038/nchembio.2329.
(33) Testa, A.; Hughes, S. J.; Lucas, X.; Wright, J. E.; Ciulli, A. Structure-Based Design of a Macrocyclic PROTAC. Angewandte Chemie – International Edition 2020, 59 (4), 1727–1734. https://doi.org/10.1002/anie.201914396.
(34) Farnaby, W.; Koegl, M.; Roy, M. J.; Whitworth, C.; Diers, E.; Trainor, N.; Zollman, D.; Steurer, S.; Karolyi-Oezguer, J.; Riedmueller, C.; Gmaschitz, T.; Wachter, J.; Dank, C.; Galant, M.; Sharps, B.; Rumpel, K.; Traxler, E.; Gerstberger, T.; Schnitzer, R.; Petermann, O.; Greb, P.; Weinstabl, H.; Bader, G.; Zoephel, A.; Weiss-Puxbaum, A.; Ehrenhöfer-Wölfer, K.; Wöhrle, S.; Boehmelt, G.; Rinnenthal, J.; Arnhof, H.; Wiechens, N.; Wu, M. Y.; Owen-Hughes, T.; Ettmayer, P.; Pearson, M.; McConnell, D. B.; Ciulli, A. BAF Complex Vulnerabilities in Cancer Demonstrated via Structure-Based PROTAC Design. Nat Chem Biol 2019, 15 (7), 672–680. https://doi.org/10.1038/s41589-019-0294-6.
(35) Chung, C. W.; Dai, H.; Fernandez, E.; Tinworth, C. P.; Churcher, I.; Cryan, J.; Denyer, J.; Harling, J. D.; Konopacka, A.; Queisser, M. A.; Tame, C. J.; Watt, G.; Jiang, F.; Qian, D.; Benowitz, A. B. Structural Insights into PROTAC-Mediated Degradation of Bcl-XL. ACS Chem Biol 2020, 15 (9), 2316–2323. https://doi.org/10.1021/acschembio.0c00266.
(36) Smith, B. E.; Wang, S. L.; Jaime-Figueroa, S.; Harbin, A.; Wang, J.; Hamman, B. D.; Crews, C. M. Differential PROTAC Substrate Specificity Dictated by Orientation of Recruited E3 Ligase. Nat Commun 2019, 10 (1). https://doi.org/10.1038/s41467-018-08027-7.
(37) Edmondson, S. D.; Yang, B.; Fallan, C. Proteolysis Targeting Chimeras (PROTACs)in ‘beyond Rule-of-Five’ Chemical Space: Recent Progress and Future Challenges. Bioorganic and Medicinal Chemistry Letters. Elsevier Ltd July 1, 2019, pp 1555–1564. https://doi.org/10.1016/j.bmcl.2019.04.030.
(38) Scott, D. E.; Rooney, T. P. C.; Bayle, E. D.; Mirza, T.; Willems, H. M. G.; Clarke, J. H.; Andrews, S. P.; Skidmore, J. Systematic Investigation of the Permeability of Androgen Receptor PROTACs. ACS Med Chem Lett 2020, 11 (8), 1539–1547. https://doi.org/10.1021/acsmedchemlett.0c00194.
(39) Pillow, T. H.; Adhikari, P.; Blake, R. A.; Chen, J.; del Rosario, G.; Deshmukh, G.; Figueroa, I.; Gascoigne, K. E.; Kamath, A. v.; Kaufman, S.; Kleinheinz, T.; Kozak, K. R.; Latifi, B.; Leipold, D. D.; Sing Li, C.; Li, R.; Mulvihill, M. M.; O’Donohue, A.; Rowntree, R. K.; Sadowsky, J. D.; Wai, J.; Wang, X.; Wu, C.; Xu, Z.; Yao, H.; Yu, S. F.; Zhang, D.; Zang, R.; Zhang, H.; Zhou, H.; Zhu, X.; Dragovich, P. S. Antibody Conjugation of a Chimeric BET Degrader Enables in Vivo Activity. ChemMedChem 2020, 15 (1), 17–25. https://doi.org/10.1002/cmdc.201900497.